Abstract
Traumatic brain injury (TBI) of varying severity can result in balance and movement disorders, for which the benefits of treatment with physical therapy has limits. In this study, patients with post-TBI balance issues received translingual neural stimulation (TLNS) in concert with physical therapy and the effects on the grey matter volume (GMV) were evaluated. TBI-related balance and movement impairments were also assessed through Sensory Organization Test (SOT) and Dynamic Gait Index (DGI) scoring. When comparing pre- and post-intervention results, the most prominent GMV changes were increases within the cerebellum, and temporal regions, which are involved in automatic processing of gait, balance, motor control, and visual-motion. Decreases of GMV in frontal, occipital lobes (involved in less automatic processing or more conscious/effortful processing of gait, balance, motor control, and vision) positively correlated to increases in SOT/DGI scores. These results indicate that TLNS can produce brain plasticity changes leading to positive changes in functional assessments. Overall, these data indicate that TLNS delivered in conjunction with physical therapy, is a safe, effective, and integrative way to treat TBI.
INTRODUCTION
The Centers for Disease Control and Prevention (CDC) report that up to 5.3 million people in the USA are living with a disability related to traumatic brain injury (TBI),1 resulting in $76.5 billion per year2 in medical and rehabilitation costs. The majority of TBI are considered mild-to-moderate (mmTBI)3 and development of balance impairments after injury is common, occurring in 30% to 65% of patients.4 Currently, the main approach for treating mmTBI symptoms is physical therapy (PT), but its effect has limits and improvements in function are often lost if therapy is not sustained.5 Recent studies have demonstrated the effective outcomes with motor-behavioural interventions and cognitive skill training after injury,6-9 which support the development of a unified and multidisciplinary approach to mmTBI treatment.10-12
A treatment plan utilising translingual neural stimulation (TLNS) combines electrical stimulation of cranial nerves V (trigeminal) and VII (facial) with physical therapy mainly aimed at restoring balance and gait.13 TLNS can be provided via the Portable Neuromodulation Stimulator (PoNS®, Helius Medical Technologies, Newtown, Pennsylvania, USA), an investigational medical device that delivers sequenced patterns of electrical stimulation on the tongue. These stimuli then trigger the trigeminal and facial nerves to excite a natural flow of neural impulses to the brainstem or cerebellum and promote changes in targeted brain structures.14-16 Results from pilot studies of TLNS treatment of patients after mmTBI suggest that, in the absence of identifiable tissue damage, a combination of neurostimulation and rehabilitation that is both targeted and challenging will induce neuroplastic changes (i.e., brain regions of pons, brainstem, and cerebellum), reduce symptoms, and begin normalising function.13,14,16,17 In TLNS, stimulation of the tongue can occur with either a high-frequency (HFP) or low-frequency (LFP) pulse device. A long-term clinical trial was recently completed to investigate the efficacy of TLNS in patients with mmTBI symptoms and compare outcomes of the HFP and LFP devices.18
To better understand the effects of TLNS, the substudy presented here was developed with the primary objective of using structural MRI (sMRI) to evaluate cortical and subcortical changes in the brains of patients before (pre-) and after (post-) treatment. Specifically, the grey matter volume (GMV) results before and after treatment were compared to investigate if individuals experienced a reduction in compensatory brain regions and, conversely, growth in deficient automatic brain regions. These structural changes were then correlated to behavioural assessments of balance and gait before and after treatment.
METHODS AND MATERIALS
Participants
Participants were recruited through print and radio advertising and were required to have mmTBI that occurred 1 year before enrolment, reached a functional plateau in their recovery (as defined by a discharge note from their physical therapist), and a NeuroComa Sensory Organization Test (SOT) composite score 16 points below normal after adjustment for age. Mild and moderate TBI diagnoses were made based on guidelines established by Veterans Affairs/Department of Defense.19 All participants had a nonremarkable neuroradiographic report after their most recent TBI, meaning that the findings were not significant per the clinical judgement of the neuroradiologist. Reports were reviewed to rule out refractory subdural haematomas, evidence of tumours, anatomical anomalies, or evidence of loss of grey matter. Neuroradiographic reports and therapy discharge notes were obtained through a medical records request; MRI prior to enrolment was required if a participant lacked a neuroradiographic report. Potential participants were excluded if they had oral or other health problems that would preclude TLNS or, in the opinion of the investigators, were unable to successfully complete the stimulation intensity level setting procedure for the device. Additional inclusion and exclusion criteria are available (please see next paragraph for the detailed criteria for inclusion and exclusion about all the nine mmTBI participants who received HFP or LFP stimulation). Rolling recruitment was used, and enrolled participants had a unique 3-digit identifier that was used for double blinding and 1:1 randomisation by a clinical monitor.
Nine participants with mmTBI (at least 1 year post-injury) were involved (age range: 43–62 years; mean age: 53.11; standard deviation: 6.60; 6 female and 3 male) in the study. The Institutional Review Board (IRB) at School of Medicine and Public Health, University of Wisconsin–Madison, Madison, Wisconsin, USA, approved all aspects of this study, and all the nine participants were recruited on a voluntary basis, signed the approved consent form before beginning of the study. They completed consent, screening, and informational forms during their first visit and the informed written consent was obtained from all participants. All participants who chose to enrol in the TBI study were also offered the opportunity to enrol in the MRI substudy.
Moreover, all nine participants received HFP or LFP stimulation, and the detailed criteria for inclusion and exclusion were: participants able to walk independently for at least 20 minutes, had access to a treadmill while not in the clinic, and had no changes in their medications for at least 3 months prior to participation Exclusion criteria included oral health problems, nonremovable metal orthodontic devices, or oral cavity piercings that could interfere with TLNS use, chronic infectious disease, unmanaged hypertension, diabetes, neurological disorders other than those attributed to their primary diagnosis, history of treatment for cancer other than basal cell carcinoma within the past year, a penetrating head injury, craniotomy, or refractory subdural haematoma. Long-term use of psychoactive or psychostimulant medications that, in the opinion of the investigators, would compromise the participant’s ability to comprehend and perform study activities was also grounds for exclusion, as was the presence of a pacemaker or elevated risk for cardiovascular events. Individuals with a lower extremity biomechanical prosthetic, history of seizures, or a ‘severe’ score in any of the attention, memory, or executive functions categories on the Cognitive Linguistic Quick Test (CLQT) were also excluded.20
Intervention
The intervention TLNS training programme focussed on balance and gait, and consists of twice-daily in-lab training for 2-weeks (with at-home training during the intervening weekend). The participants also received physical exercise training to develop improved motor coordination and mobility as part of the TLNS training. All participants returned to the clinic weekly during the at-home phase for a single session of retraining and progression, and participated in periodic retesting. Multiple assessment metrics would capture data at the beginning and end of the 2-week in-lab TLNS intervention period and at 3-week intervals. sMRI was performed before the first intervention (‘pre’) and then 4–6 hours after the training session (‘post’) of the TLNS intervention. Overall, five patients received HFP and four patients received LFP stimulation.
Behavioural Testing
SOT is an objective, automated measure of sensory-motor integration that evaluates the functional contribution of the somatosensory, visual, and vestibular components of balance. All participants were tested SOT on the NeuroCom® Computerized Dynamic Posturography (CDP) before and after the week of twice-daily interventions.
Dynamic Gait Index (DGI) is a clinician-scored index of 8 facets of gait. Scores range from 0 (worst) to 24 (normal). A score change of 3.0 is generally considered clinically significant. The DGI scores indicate significant improvements in stability and gait that are retained for as much as 6 hours after completion of the second intervention session in the day.
MRI Acquisition
sMRI scan (3T MRI GE750 scanner, GE Healthcare, Waukesha, Wisconsin, USA) was performed before the intervention, and was also performed immediately after the intervention. T1-weighted axial anatomical scans were acquired using 3D fast spoiled gradient echo recalled brain volume (FSPGR BRAVO) sequence (repetition time: 8.132 ms; echo time: 3.18 ms; time of inversion: 450 ms) over a 256 x 256 matrix and 156 slices (flip angle: 12°; field of view: 25.6 cm; slice thickness: 1 mm). During the scanning, patients laid supine on the scanner bed and were instructed to close their eyes and keep their heads still to relax.
Data Preprocessing and Statistical Analysis
The preprocessing and statistical analysis for GMV was applied through the toolbox of the CAT1221 that works together with Statistical Parametric Mapping (version 12, Wellcome Trust Centre for Neuroimaging, London, UK);22 as well as with MATLAB (MathWorks, Natick, Massachusetts, USA).23 For the preprocessing, brain tissue was segmented into grey matter, white matter, and cerebrospinal fluid using the segment procedure. Images were transformed nonlinearly to standard stereotaxic space (Montreal Neurological Institute, Montreal, Canada) and resliced to 1.5 × 1.5 × 1.5 mm using the diffeomorphic registration algorithm (DARTEL).24-27 Grey matter probability maps were then multiplied by the non-linear component of the Jacobian determinant. Finally, modulated grey matter probability maps were spatially smoothed with an 8 mm full-width at half-maximum Gaussian kernel.
For the statistical analysis, the paired t-test (before versus after interventions) was used for calculating the GMV and behaviour score, respectively. The statistical threshold was set to p<0.05 and cluster size >212 using the AlphaSim correction28-30 for GMV, and was set to p<0.05 for behaviour score (SOT or DGI in each) with IBM SPSS version 23. Moreover, the correlation analysis between GMV (post- minus pre-) and SOT (or DGI; post- minus pre-) was set to correct p<0.05 with IBM SPSS version 23.
RESULTS
There was a significant increase from pre- to post-intervention for both mean SOT (t(8)= 2.74; p=0.03) and DGI (t(8)= 2.86; p=0.02) based on the paired t-test calculations.
There were also significant changes in GMV in specific brain regions when comparing pre- versus post-treatment (Table 1 and Figure 1). A positive t-value represents a decrease in GMV post-, compared to pre-treatment, and the converse is represented by a negative t-value.
Compared to pre-, post-treatment there were decreases in GMV in nine specific regions (eight of these in the frontal and parietal lobes) and increases across 11 regions of the temporal, frontal, and occipital lobes and cerebellum. A second analysis, employing a Gaussian random field correction, had the same trend for increases and decreases in GMV in the regions noted above.
There were some significant negative correlations between GMV and SOT or DGI (post- minus pre-intervention). The SOT showed all negative correlations to the frontal regions, and the DGI showed all negative correlations to the frontal (right superior frontal gyrus, left superior medial frontal gyrus) and occipital (right cuneus) regions (Table 2). However, no positive correlations were observed between GMV and either SOT or DGI.
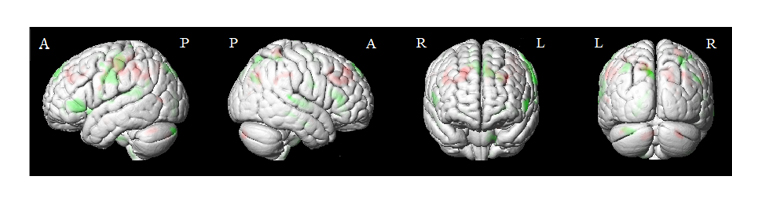
Figure 1: The paired t-test of grey matter volume before (pre-) versus after (post-) treatment. The grey matter volume statistical threshold was used with AlphaSim corrected p<0.05, cluster size >212.
Red: pre- was increased than postintervention.
Negative value: pre- was decreased than postintervention.
Parts of brain: A: anterior; P: posterior; L: left; R: right.
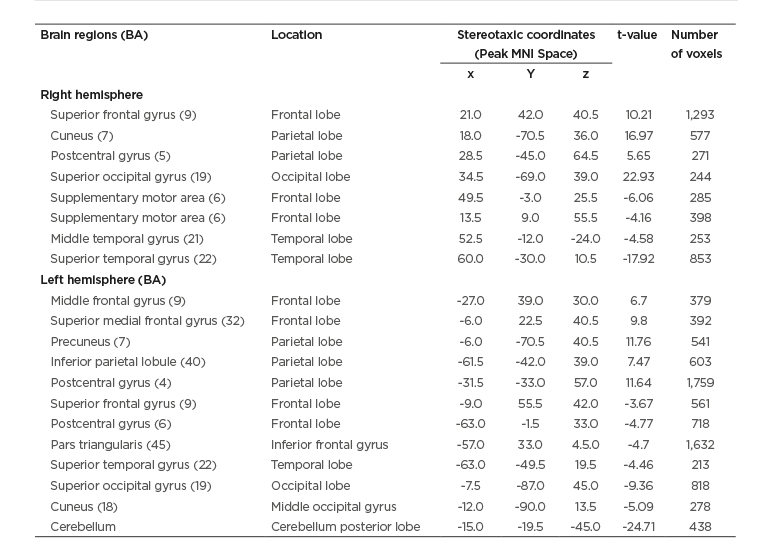
Table 1: The paired t-test of grey matter volume before (pre-) versus after (post-) treatment.
The grey matter volume statistical threshold was used with AlphaSim corrected p <0.05, cluster size >212.
Positive value: pre- was increased than postintervention.
Negative value: pre- was decreased than post-intervention.
BA: Brodmann area; MNI: Montreal Neurological Institute.
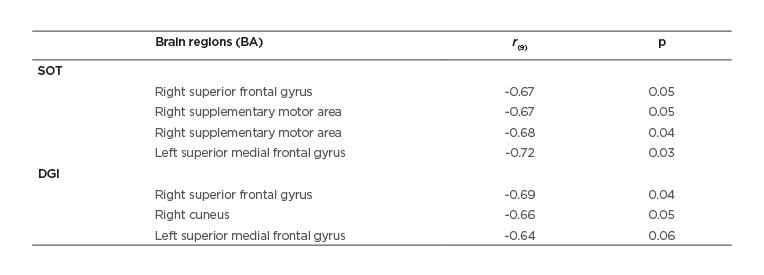
Table 2: Correlations between grey matter volume (post- minus pre-) and behaviour testing (post- minus pre-).
The statistical threshold was set to corrected p<0.05 with SPSS16.0.
DISCUSSION
This substudy of a randomised controlled clinical trial reports notable differences in brain structure in mmTBI patients after TLNS treatment.
The authors observed that there were increases of brain volumes within the temporal, frontal, and occipital lobes, as well as decreases in volumes within the frontal and parietal lobes, and statistically significant improvements in SOT and DGI assessments post- versus pretreatment. Specifically, the cerebellum had the largest increase in GMV (t= -24.71) after therapeutic intervention. The cerebellum is an important junction in the control of balance, because it coordinates information from the vestibular system, the cerebral cortex, and muscles and joints, in order to aid body adjustments and control balance.31 The second highest increase in GMV was in the right superior temporal gyrus (t= -17.92), where activation during balance exercises has been previously reported32 and may relate to the role of this region in visual–motion processing.
A decrease in GMV after treatment was calculated for several regions, including those associated with sensorimotor processing, visual processing, motor imagery, working memory, and executive function (i.e., right superior frontal gyrus, right cuneus, and left precuneus),33-36 with the largest decrease observed in the right superior occipital gyrus which is responsible for visual attention37 or control monitoring.38 The superior medial frontal cortex also had a decrease in GMV after treatment; this region has the higher-level cognitive functions such as attention, working memory, and cognitive monitoring,39 and is also involved in automatic subconscious40 and involuntary motor control.41 These defined decreases in GMV indicate a degree of structural control and change, after treatment, in brain regions involved in less conscious/automatic motor plans.
Correlative analyses between the GMV and SOT or DGI determined that all changes in GMV were negatively correlated to each assessment; therefore, higher improvements in behaviour score would be associated with less change in GMV in the areas assessed. As mentioned above, the superior frontal gyrus, superior medial frontal gyrus, supplementary motor area, and cuneus are responsible for sensorimotor processing, behavioural control or monitoring, and visual processing, and the negative correlations between the behaviour measure and brain GMV possibly reflect greater efficiency and automatised sensorimotor function after treatment. Moreover, it is speculated that in addition to the brain regions listed in Table 2, the more improved score on behavioural assessment possibly indicates the more other potential regions are involved to play the compensatory role in participants with TLNS treatment. In other words, the improved score on behaviour assessment could reflect adaptive plasticity of functional or structural connections among brain regions, which is a result of brain recovery.42
While these initial results are compelling, the small sample size of this substudy does impose two basic limitations of the analyses that should be further investigated with follow-up studies with more patients. First, many of the less significant regions (t-value <10) in GMV should be further investigated with a larger patient population to determine if a larger sample size will produce similar outcomes. Additional analyses will help support the purpose of this pilot study, which was to demonstrate, preclinically, the significance of the efficacy of TLNS stimulation to treat mmTBI. Secondly, the HFP and LFP groups were pooled for outcomes analyses; their separation will more specifically define recovery outcomes provided by the HFP device.
The adverse effects of the TLNS treatment should also be noted. The sensation during stimulation could become uncomfortable at high intensity levels, which could be distressing to the participants. In pilot studies, some participants had reported a mild tingling sensation that lasted from several minutes up to an hour after the stimulus was turned off.13 Although this sensation might feel unusual, it was not reported to be annoying and did not interfere with normal activities, because participants could preset the intensity level to within the preferred stimulus intensity range, between sensation threshold and maximum level without discomfort.13 The current study also confirmed this. Moreover, participants might experience excess salivation as a result of the presence of the PoNS in mouth, and because more than one participant would use the same device there was a potential for disease transmission. This risk was reduced to an extremely small level by three mechanisms: first, participants typically learnt to regulate saliva by learning to swallow with the device in their mouth, so this way was less a risk than a side effect; second, participants reporting transmittable diseases, or having apparent oral lesions or inflammation, would not be allowed to participate in the study; third, the electrode array would also be cold sterilised according to the protocol developed and approved by the Infection Control Office at University of Wisconsin Health Center. Furthermore, participants would be performing balance and gait tasks that might cause them to lose their balance or fall, so they were protected wherever possible. During in-lab balance training, the participants were surrounded on three sides by a custom laboratory bench designed for this purpose, while the treadmill had a handrail that surrounds the participant on three sides. Additionally, participants might be required to wear a safety belt during gait tests and training to prevent falls.
SUMMARY
Overall, these results suggest that patients may demonstrate improvements in balance and movement with increase in involvement of the neural regions involved in automatic subconscious40 and involuntary motor control, gait, and balance41 and decrease in involvement of neural regions involvement in planning, visual, executive function, or cognitive functions. Although additional information can be gained in follow-up studies, this current substudy indicated that TLNS is a safe, effective, and integrative method to treat mmTBI. The benefits from this intervention include symptom improvement that will affect the patients’ quality of life and functional capacities. These benefits far outweigh the risks associated with the study. With proper instruction and monitoring, these risks could be managed and minimised and could also improve the rehabilitation outcomes of people with chronic symptoms experienced with TBI.