Abstract
Postmenopausal osteoporosis is an oestrogen deficiency-induced, systemic skeletal disease that affects the quality of life of patients once severe complications develop. The imbalance in osteoclastogenesis and osteoblastogenesis is the crucial pathological basis of osteoporosis and it is affected by classical pathways, epigenetic regulation, post-transcriptional regulation, oxidative stress-mediated signalling, and gut microbiotas. New methods to manage postmenopausal osteoporosis are essential and urgent. Dual-energy X-ray absorptiometry derived bone mineral density is acknowledged as the gold standard for osteoporosis diagnosis, and FRAX®, along with other clinical risk factors, has been used for osteoporotic fracture assessment. Novel serum biomarkers, such as circulating microRNA, are emerging and showing potential for diagnosing osteoporosis and estimating fracture risk. A major aim of osteoporosis diagnosis is to clarify the origins of the disease, clarify the functions of biomarkers and their dynamic changes responding to therapy, and develop a novel diagnostic strategy in combination with current methods. Traditional therapeutics, including bisphosphonates, denosumab, oestrogen replacement, and teriparatide, have been used in osteoporosis therapy for a long time. Some severe side effects have resulted in therapy discontinuation; however, the incidence of adverse reactions is quite low. Developing novel treatments for osteoporosis using mesenchymal stem cells or Chinese medicinal herb-based therapy is of increasing interest to researchers, based on their improved safety, efficiency, and cost performance. Improvements in both diagnostic and therapeutic strategies may contribute to personalised management of osteoporosis.
EPIDEMIOLOGY
Osteoporosis is a systemic skeletal disease characterised by microarchitectural deterioration and high fragility of bone tissue, resulting in low bone mineral density (BMD) and poor bone quality, generally ascribable to oestrogen deficiency or ageing.1 Osteoporosis is commonly regarded as a silent disease until it is complicated by systemic pain, spine deformation, height reduction, and fragility fracture. Fragility, or osteoporotic, fracture is the most dangerous complication of osteoporosis. These fractures can occur following minor trauma, but they can also occur spontaneously.1 In the skeletal system, the spine, hip, and distal forearm are the regions most susceptible to osteoporotic fractures. In the USA, >9.9 million people have been diagnosed with osteoporosis and 43.1 million people are in a state of osteopenia.2,3 Among them, nearly 1.5 million patients experience fragility fractures each year.2,3 In Europe, 27.6 million people are diagnosed as osteoporotic each year, with >3.5 million of them experiencing osteoporotic fractures each year.3,4 Until 2006, approximately 69.4 million people >50 years old were estimated to have osteoporosis, and 2.1 million people had osteopenia in China.5 According to an epidemiological investigation of the population of Beijing, the incidence of spine fracture was about 15% in women >50 years of age.5 In addition, the incidence of hip fractures had sharply increased by 42% in men and 110% in women from 1990–1992 compared with 2002–2006.5 Fragility fracture augments the disability and mortality of osteoporotic patients; for example, about 20% of fragility fracture patients died of various complications within 1 year of hip fracture, while about half of the survivors who sustained a hip fracture remained disabled.5 All of the aforementioned factors impose a great socioeconomic burden worldwide. Since oestrogen deficiency represents the most common cause of osteoporosis, this article will concentrate on the topic of postmenopausal osteoporosis.
PATHOPHYSIOLOGY OF POSTMENOPAUSAL OSTEOPOROSIS
The critical role of oestrogen deficiency in the pathogenesis of osteoporosis is based on the fact that postmenopausal women are at the highest risk of developing the disease (Figure 1). The bone metabolism of postmenopausal women is characterised by high bone turnover, which is defined as coinstantaneous increase of bone resorption and bone formation.6 However, bone resorption exceeds bone formation after menopause, leading to an imbalance of bone remodelling and a rapid net bone loss.6 In the first 5 years after menopause, bone loss occurs drastically and primarily in cancellous bone, while in the later years the bone mass decreases more slowly and mainly affects cortical and trabecular bone space, a process that can last for >10 years.7
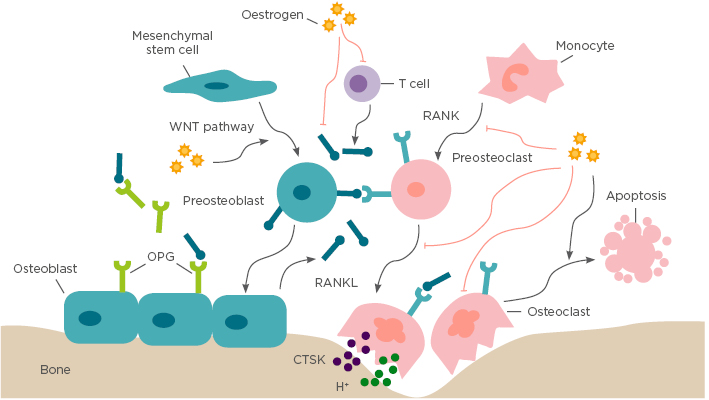
Figure 1: Bone remodelling.
Bone remodelling is based on a balance between osteoclastogenic bone resorption and osteoblastogenic bone formation, which is a crucial dynamic procedure during bone growth, development, and regeneration. Osteoblasts stem from mesenchymal stem cells and specifically generate extracellular bone matrix through the WNT signalling pathway. Osteoclasts originate from monocytic lineage and secrete bone resorptive factors through the RANK/RANKL/OPG signalling pathway. In addition, oestrogen also regulates bone remodelling by suppressing RANKL expression by T cells, mesenchymal stem cells, and osteoblasts. CTSK: cathepsin K; OPG: osteoprotegerin; RANK: receptor activator of NFκB; RANKL: receptor activator of NFκB ligand.
Under physiological conditions, continuous and harmonious bone remodelling is maintained by an organised sequence of bone resorption followed by bone formation, which is termed osteoclast–osteoblast coupling. This partnership develops in response to dual regulation of mechanical force and endocrine factors.8 Corporation of calcium and type I collagen-based bone matrix is crucial for osteoblast-mediated bone formation, which is under the control of endocrine factors, especially oestrogen.8 Oestrogen has a central role in normal physiological remodelling; oestrogen deficiency breaks the balance of osteoclastogenesis and osteoblastogenesis, resulting in progressive bone loss (Figure 1).
The oestrogen receptors (OR) ORα and ORβ have been detected in osteoblasts, osteoclasts, and osteocytes. The nuclear receptor, ORα, primarily regulates bone metabolism.7 Once bound to its ligand, ORα recruits coactivators or corepressors, and modulates transcription of oestrogen-responsive target genes accordingly. In addition, ORα can interact with transcriptional factors, including NFκB, and, as a result, downregulate its own downstream signals.9 Osteoblasts and osteoclasts are involved in changes of cell fate in response to oestrogen deficiency, such as proliferation, differentiation, programmed cell death, and altered expression of target genes.
It is well known that osteoclast progenitors express receptor activator of NF κB (RANK), while osteoblasts express the ligand of RANK (RANKL) and the RANK antagonist osteoprotegerin (OPG). Physiologically, oestrogen regulates osteoclastogenesis via the core RANK/RANKL/OPG signalling pathway both in vitro and in vivo.10,11 In the absence of oestrogen, enhanced crosstalk between RANKL and RANK provides the pivotal signal to promote osteoclast maturation and activation of osteoclast function.12 Additionally, a reduced antagonism of RANKL by OPG also facilitates osteoclast formation and activation. Furthermore, an inflammatory microenvironment has been reported to be a crucial factor for oestrogen-mediated osteoclastogenesis regulation, for example, oestrogen maintains bone remodelling balance by enhancing osteoclasts apoptosis mediated by increased production of TGF-β.13 However, in an oestrogen deficient environment, formation of osteoclasts is accelerated by increased osteoclastogenic proinflammatory cytokines, including IL-1, IL-6, IL-17, and TNF-α, which are negatively regulated by oestrogen.14
Bone morphogenetic proteins (BMP), TGF-β, insulin-like growth factor 1 receptor, and the WNT pathway are well-acknowledged, crucial signals involved in osteoblastogenesis regulation and bone formation, which are directly or indirectly regulated by oestrogen.15 Oestrogen positively regulates osteoblastogenesis by stimulating the production of pro-osteoblastogenic factors, such as BMP, TGF-β, fibroblast growth factors, insulin-like growth factor 1, parathyroid hormone, and procollagen, resulting in the promotion of osteoblast formation, activity, and lifespan.16
Recently, pluripotent stem cells have been reported to be crucial in the regulation of the function and regeneration of local tissues. Mesenchymal stem cells (MSC) are commonly regarded as osteoblast precursors, which are critical for maintaining the balance of bone formation and resorption or the balance of bone formation and adipose formation.17 Osteogenic differentiation of MSC is regulated by complex signalling. For instance, functional defects of the ALP, ERK, and FAS pathways;18 epigenetic regulatory enzyme EZH2;19 and GCN520 cause MSC dysfunction, sequential bone metabolism imbalance, and final osteoporosis. Disorders of MSC are largely considered the key pathological factor in the development of oestrogen deficiency-induced bone loss.21 Oestrogen-deficiency induces chronic inflammation (via the promotion of TNFα, IFN-γ, IL-1, and IL-6 activity) that blocks MSC function and further initiates osteoporosis.14 Moreover, during the osteoporotic process, microRNA (miRNA) play a significant role in the regulation of MSC function at the post-transcriptional level. Oestrogen deficiency alters MSC microRNA profile. microRNA, such as let-7, miR-17,22 miR-26a,23,24 miR-181a,25 miR-705, and miR-3077,26,27 can positively or negatively regulate osteogenic differentiation of MSC and osteoporosis development.
In addition, oxidative stress has been proposed as an alternative aetiology of osteoporosis for about two decades.28 Elevation of reactive oxygen species following oestrogen deficiency enhances osteoblasts apoptosis and blocks the MSC survival and their functions.29 Excessive reactive oxygen species in postmenopausal osteoporosis can largely be ascribed to the activation of the pro-oxidant enzyme system and deterioration of the enzymatic antioxidant system. NADPH oxidase 4 was observed to be provoked, meanwhile manganese superoxide dismutase and catalase were decreased during postmenopausal osteoporosis.28,30,31 Rebalancing the pro-oxidant and antioxidant system effectively prevents oestrogen deficiency-induced bone loss.
Recently, the gut microbiota has been investigated as a crucial regulator of bone metabolic disorders in osteoporotic diseases. A germ-free mouse model, housed in a sterile environment, was used to understand the relationship between the microbiota, bone health, and systemic asepsis.32 The germ-free mice showed higher bone density compared with mice raised in conventional conditions, suggesting that the microbiome was closely related to bone health.32 Aberrant gut microbiota resulted in bone loss, whereas probiotic or antibiotic administration was demonstrated to rescue bone degeneration through modulating immune system, absorption of intestinal calcium in oestrogen deficiency-induced osteoporosis.32 CD4+ T cells and inflammatory factors are commonly regarded as promoters of osteoclastogenesis during oestrogen deficiency.33 A large decrease in CD4+ T cell populations and inflammatory factors was observed in the bone marrow following a treatment with probiotics.32 However, the effects of the gut microbiota on bone health are complex and the precise mechanisms remain elusive.
DIAGNOSIS
Indications for Osteoporosis Diagnosis
Osteoporosis is a silent disease. Most osteoporotic patients do not realise they have the condition until its severe complications occur. Gynaecologists or family medicine physicians are usually the first healthcare providers who face the problem; therefore, indications for suspecting osteoporosis are necessary for them to decide whether the female patients should be sent for BMD testing. The US National Osteoporosis Foundation (NOF) has made the following recommendations, highlighting those most at risk 1) women >65 years of age, regardless of clinical risk factors; 2) younger postmenopausal women and women in the menopausal transition; 3) adults who have a fracture after 50 years of age; and 4) adults with a skeletal disease or taking a drug associated with low bone mass.
Traditional Diagnostic Regimen
BMD-derived, normalised, T score of the 1–4 lumbar vertebras, hip, or femoral neck is commonly accepted as the international gold standard for diagnosis of osteoporosis based on dual energy X-ray absorptiometry (DXA). T score is calculated as the formula: (BMD of candidate – peak BMD of population of the same sex)/standard deviation of peak BMD of population of the same sex. Candidates with T score ≥-1.0 are diagnosed as healthy; T scores between -2.5 and -1.0 are diagnosed as osteopenic; and T score ≤-2.5 are diagnosed as osteoporotic.1
BMD is the basis of future fracture risk assessment and, since the early 1990s, it has been well reported to be negatively related to the risk of future fracture incidence.34 However, it is imprecise to evaluate fracture risk using BMD alone because of the fact that osteoporotic patients with a similar BMD sometimes show different fracture risks. Therefore, the World Health Organization (WHO) Fracture Risk Algorithm (FRAX®)35 was developed based on femoral neck BMD and the clinical risk factors (current age, sex, a prior osteoporotic fracture, low BMI, rheumatoid arthritis, secondary causes of osteoporosis, parental history of hip fracture, smoker status, alcohol intake, and oral glucocorticoids) to estimate the 10-year probability of a major osteoporotic fracture.35 However, this is not a rule, rather a clinical guideline; all management decisions should be made in consideration of clinical judgment on a case-by-case basis. Thus, consideration of the internal and external risk factors independent of BMD has been proposed by the WHO to improve the assessment strategy of osteoporotic fracture risk. Several risk factors have been identified, including lifestyle factors (low calcium supplement, excessive thinness, immobilisation, and falling), genetic diseases, endocrine disorders, hypogonadal states, rheumatologic and autoimmune diseases, gastrointestinal disorders, haematological diseases, neurological and musculoskeletal disorders, miscellaneous diseases, and pharmaceutical intervention.1
Serum and urine bone turnover biomarkers have been developed to estimate the status of bone formation and bone resorption, which are generally non-invasive and highly cost-effective. The most widely available markers include serum osteocalcin; bone-specific alkaline phosphatase; and total N-terminal propeptides of type I procollagen (tPINP), which are markers of bone formation; and urine or serum C-terminal cross-linking telopeptides of type I collagen (CTX-1) and tartrate-resistant acid phosphatase, which are markers of bone resorption.1,36 Serum tPINP and CTX-1 are recommended for osteoporosis auxiliary diagnosis by the International Osteoporosis Foundation (IOF).6 A correlation between osteoporotic status/fractures and bone turnover biomarkers independent of BMD has been reported in the osteoporotic population, including postmenopausal women.6 Although the bone turnover markers have been developed for decades, their accuracy, specificity, sensitivity, and stability are still undefined in the clinical approach to osteoporosis management.
New Diagnostic Regimen
Recently, circulating microRNA have emerged as novel biomarkers for osteoporosis diagnosis and fracture prediction. Circulating microRNA are a type of small RNA that exist in body fluid, which are always specifically expressed in vivo and maintain stability for a long time in vitro.37 Compared with traditional serum bone turnover markers, circulating microRNA have the advantages of higher sensitivity, stronger specificity, and better stability. It has been reported that dysregulated expression of circulating microRNA is always closely related to the pathological physiology of diseases.38-40 Therefore, circulating microRNA has potential as a molecular diagnostic biomarker in the clinic.41 According to research with a sample size of 120, serum miR-21 and miR-133a exhibited high potential as diagnostic markers for osteoporosis.42 The researchers observed that serum miR-21 was downregulated among healthy participants, osteopenic patients, and osteoporotic patients; the opposite was true for miR-133a. In addition, miR-21, miR-23a, miR-24, miR-25, miR-100, miR-125b, miR-382-3p, miR-550a-5p, miR-122-5p, and miR-125-5p were also reported as potential predictors of osteoporotic fracture.38,43,44 Moreover, a combinative panel of nine circulating microRNA (including miR-942-5p, miR-155-5p, miR-330-3p, miR-203a, and miR-181c-5p) showed a satisfactory predictive performance with an area under the curve of 0.97 to estimate osteoporotic fracture.45
However, the limitations of these biomarkers cannot not be neglected. The biomarkers are limited by a number of factors, such as easy degradation, temporal variability, the influence of food intake.36 Despite these drawbacks, three additional points may yet come to influence the prospect of biomarkers application for osteoporosis: 1) clarify the origin of biomarkers and whether biomarkers function during osteoporosis development; 2) monitor dynamic changes to biomarkers to estimate the effects of anti-osteoporotic therapy; and 3) develop higher effective diagnostic strategy combined with traditional measures. Personalised diagnosis and treatment might be feasible in the future to manage osteoporosis through combined application of BMD, clinical risk factors, and disease-specific biomarkers.
THERAPEUTICS
Traditional Treatments
At present, the most common approach to preventing and treating osteoporosis is osteoclast inhibition. Several antiresorptive pharmaceuticals approved by the U.S. Food and Drug Administration (FDA) have been applied to osteoporosis treatment. Bisphosphonates, including alendronate, ibandronate, risedronate, and zoledronic acid, are antiresorptive drugs, which specifically bind to hydroxyapatite in bone tissues and sequentially inhibit osteoclasts attachment to bone surface, and through the suppression of lysosomal enzyme, pyrophosphatase, and prostaglandin, among proteins, prevent bone resorption.46 Bisphosphonates have been widely used; the drug group represents a great advance in the treatment of osteoporosis and prevention of fractures, with a reduction of about 50% in the risk of vertebral fractures, while the reduction of nonvertebral fracture has shown a very variable range.47,48 However, several side effects associated with the use of bisphosphonates have been reported, such as gastrointestinal disorders, osteonecrosis of the jaw, eye inflammation, atypical femur fractures, and renal function impairment.1 Consequently, the consensus is growing to recommend administering bisphosphonates at a low dose in weekly formulations, and the responses of BMD and bone turnover markers are no different compared to those of daily formulations.49,50
Denosumab is a monoclonal antibody with high specificity and affinity to the receptor activator of RANKL.51 It showed a prominent efficacy on postmenopausal osteoporosis with an incidence reduction of facture by approximately 70%, 40%, and 20% in vertebral, hip, and non-vertebral fractures, respectively, over 3 years.51 Additionally, denosumab has also been reported to improve BMD in men at high risk of fracture. However, an abstinence reaction with rapid increase of bone turnover markers has been observed when denosumab administration is stopped.52,53 Furthermore, the incidence of fragility fractures was back to baseline during the off-treatment stage.52,53 Therefore, alternative agents should be applied in systematic treatment in osteoporotic patients to prevent denosumab deprivation-related bone loss.
Oestrogen replacement is the symptomatic treatment of choice to rescue postmenopausal bone loss and to reduce the risk of osteoporotic fracture. The Woman’s Health Initiative (WHI) indicated that the risk of central skeleton fractures was reduced by approximately 30% and peripheral skeleton osteoporotic fractures by around 20% with a 5-year oestrogen replacement therapy regimen.1 However, the WHI also reported increased risks of cardio-cerebral vascular incident, invasive breast cancer, deep vein thrombosis, and pulmonary emboli after long-term use of oestrogen replacement therapy. Additionally, an abstinence reaction with steep bone loss follows oestrogen deprivation. These serious side effects result in low enthusiasm for oestrogen replacement application in osteoporosis therapy. Nevertheless, oestrogen replacement is still an option for postmenopausal women with a high risk of fracture.54 In addition, a selective oestrogen receptor modulator, especially raloxifene, reduces the risk of prior vertebral fractures by about 55% and vertebral refractures by about 30% over 3 years, whereas it has no effect on nonvertebral fractures.1 However, the safety of raloxifene is also a concern, with an increased risk of thromboembolic disease, stroke, hot flashes, and leg cramps.1
Teriparatide, also known as the N-terminal 34 amino acid of parathyroid hormone, activates bone resorption and bone formation simultaneously.55 It reduces the risk of vertebral fractures and non-vertebral fragility fractures by about 65% or 50% in osteoporotic patients, respectively.1 However, it has been reported to cause side effects like hypercalcaemia, nausea, and dizziness in human studies, and even osteosarcoma in animal studies.1
Therefore, it is urgent to develop safer, more effective alternative with an improved cost-effectiveness for the management of osteoporosis in consideration of adverse events and long-term safety concerns in clinical use about current therapeutic strategies.
NEW TREATMENTS
Since functional defects in MSC is the crucial aetiology for osteoporosis development, researchers have tried to treat osteoporosis through local or target transplantation of normal MSC. A study showed that bone marrow injection of bone marrow-derived mesenchymal stem cells (BMMSC)-alginate hydrogel mixture significantly improved bone deposition, hardness, and volume of trabecular bone in oestrogen deficient rabbits.56 Additionally, the cancellous bone mass of ovariectomised rats were increased after infusion of BMMSC into bone marrow.57 BMMSC coupling LLP2A has also been reported to enhance bone formation and finally increase bone mineral density in both oestrogen deficiency-induced osteoporosis and age-related osteoporosis.17 Moreover, BMMSC transplantation has also been applied to relieve MSC dysfunction-related osteodysplastic diseases. Multiple clinical trials indicated that BMMSC transplantation increased bone growth and quality, and decreased fracture incidence of patients with osteogenesis imperfecta.58 MSC transplantation ameliorates osteoporosis not only by providing normal stem cells, but also the modulation of the host’s microenvironment and improving osteogenic function of the host’s MSC. Systemic administration of MSC promotes osteogenesis of BMMSC and finally rescues the osteoporotic bone loss in oestrogen deficiency,59 glucocorticoid,60 diabetes,61 and systemic lupus erythematosus models.62 In addition, local application of cell aggregates of MSC sharply decreased the incidence of femur osteoporotic fracture from 93.75% to 37.50% in ovariectomised rat models.63 Furthermore, exosomes derived from normal MSC also exhibits satisfactory efficacy on osteoporosis treatment, which ascribes to signalling substances contained in exosomes like functional proteins, small RNA, and so on.18 Thus, MSC based therapy is a promising candidate regimen for osteoporosis treatment.
In recent years, an increasing enthusiasm has been generated by the management of osteoporotic diseases with traditional Chinese medicine as novel alternatives because of fewer adverse events and the accumulated experience of thousands of years of medicinal use (Table 1).
Chinese medicinal herbs containing multiple components usually exert their therapeutic effects on postmenopausal osteoporosis through complicated mechanisms. Multiple pathways have been revealed to rebalance osteoblastogenesis and osteoclastogenesis, including oestrogen receptor dependent, RANK/RANKL/OPG, BMP, Wnt/β-catenin, ERK, TGF-β, and Notch signalling pathways.74,75 Apart from restoring the balance between osteoblastogenesis and osteoclastogenesis, Chinese medicinal herbs have also been founded to modulate the balance of adipocytes and osteoblasts, which is also critical to bone metabolism.75 Moreover, many Chinese medicinal herbs have chemical structures or chemical groups similar to oestrogen. They exert oestrogen-like functions as well as immunoregulation and antioxidation, which have been indicated to ameliorate osteoporosis.75 In addition, Chinese medicinal herbs and other natural small molecules, like melatonin,59 rapamycin,76 and licochalcone A,63 have also been used in MSC pretreatment to improve MSC-based therapy in osteoporotic diseases. It seems feasible and valuable to develop novel therapeutics for osteoporosis therapy based on combined use of MSC and Chinese medicinal herbs.
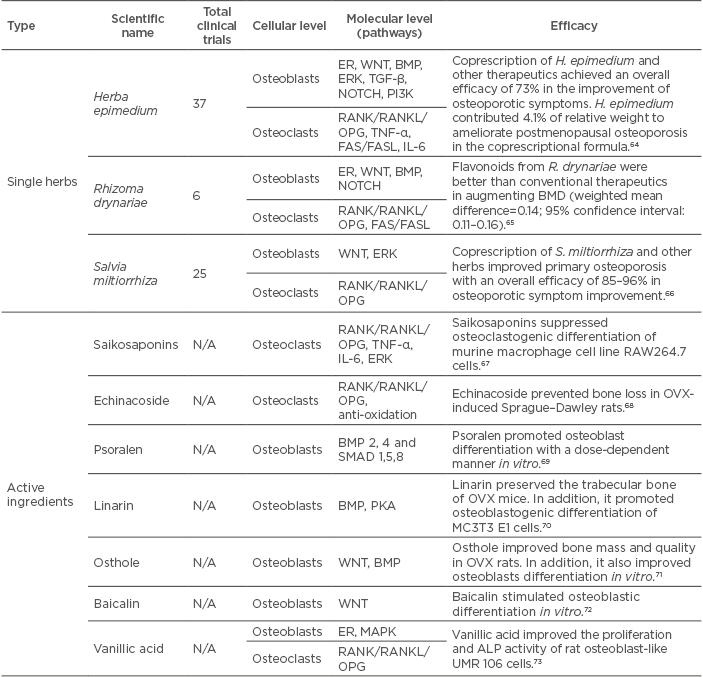
Table 1: Research on Chinese medicinal herbs on postmenopausal osteoporosis.
ALP: alkaline phosphatase; BMD: bone mineral density; BMP: bone morphogenetic protein N/A: not applicable; OPG: osteoprotegerin; PI3K: phosphatidylinositol-4,5-bisphosphate 3-kinase; PKA: protein kinase A; RANK: receptor activator of NFκB; RANKL: receptor activator of NFκB ligand; TGFβ: transforming growth factor beta.
THE FUTURE: GOAL-GUIDED TREATMENT
The current consensus is growing to manage osteoporosis, with the aim to approach target efficacy, such as target BMD or lowered fracture risk, which is termed as goal-guided treatment strategy. It is recommended to choose drugs based on the osteoporotic patient’s characteristics and therapeutic features and adopt a corresponding method to monitor therapeutic effects. However, standard treatment goals have yet to be defined. Temporarily, dynamics of BMD and bone turnover markers during the course have been proposed to guide when to pause or switch therapeutics. Apart from traditional managements, novel biomarkers and new medications might also contribute to treatment modification and further promote personalised diagnosis and therapy for osteoporosis. A synopsis of clinical management for postmenopausal osteoporosis are displayed in Table 2.
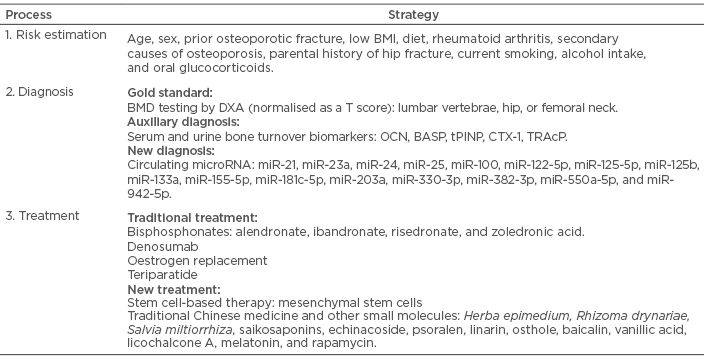
Table 2: Synopsis of clinical management for postmenopausal osteoporosis.
BASP: serum bone-specific alkaline phosphatase; BMD: bone mineral density; CTX: carboxy-terminal collagen crosslinks; DXA: dual-energy x-ray absorptiometry; OCN: osteocalcin; tPINP: type 1 N-terminal peptide; TRAcP: tartrate-resistant acid phosphatase.