Abstract
Chronic obstructive pulmonary disease (COPD) is a leading cause of death. The prevalence of the disease and associated mortality continue to increase. Bacterial and viral infections are responsible for the transition of the disease to more severe stages, resulting in COPD exacerbation. Biofilms, communities of micro-organisms that contribute to COPD exacerbation, pose a formidable challenge for effective pharmacotherapy. This review focuses on the development of biofilms, and approaches to inhibit and eradicate biofilms.
Key Points
1. Combination therapies are beneficial in eradicating biofilms; however, they pose an increased risk of side effects, allergies, toxicity, and Clostridium difficile infections. To address the issue of toxicity often seen with dual antimicrobial therapy, topical administration of these agents is recommended. Topical administration delivers high concentrations to the target site of infection with nearly undetectable serum concentrations, thereby reducing the risk of systemic side effects.
2. Use of bacteriophages as anti-biofilm agents is showing promise. Bacteriophages can prevent biofilms from forming, and can eradicate existing biofilms. Phages work by utilising enzymes to dissolve the outer matrix of the target biofilm, and once they penetrate the tough matrix, they continue to kill the bacterial cells embedded within the host biofilm.
3. In respiratory infections involving biofilms, many clinicians choose inhaled formulations of antimicrobial agents. Inhaled antimicrobials are beneficial because they allow for a higher concentrated dosage to reach the respiratory tract. While inhaled formulations limit systemic exposure of antibiotics, it is difficult to treat biofilms with single agent antimicrobials, because biofilms can survive 1,000-fold higher concentrations of antimicrobials compared to planktonic bacteria.
CHRONIC OBSTRUCTIVE PULMONARY DISEASE EXACERBATION
Chronic obstructive pulmonary disease (COPD) is the third leading cause of death globally. COPD encompasses two clinical phenotypes: emphysema and chronic bronchitis. Factors that contribute to the onset of COPD include genetic factors, pollution, cigarette smoke, and exposure to diverse chemicals. Exacerbation of COPD correlates with bacterial colonisation and respiratory viral infections. The main bacterial pathogens implicated in COPD exacerbation include Pseudomonas aeruginosa, Moraxella catarrhalis, Haemophilus influenzae, and Streptococcus pneumoniae. An important contributing factor to the virulence of bacteria associated with COPD exacerbations is their ability to form biofilms.
Micro-organisms that form biofilms pose significant health challenges attributed to human and animal infections they cause. The host immune system has difficulty in eradicating the microbial complex communities, resulting in increased antimicrobial resistance. Proinflammatory cytokines implicated in the host immune response to biofilm-forming P. aeruginosa strains include IL-6, IL-8, IL-10, TNFα, and IL-1β.1
Pathogenic bacteria can survive in the lungs of patients with COPD for several months. This may be attributed to the formation of biofilms, which may also be responsible for recurring episodes of acute exacerbations.2 Exacerbations of COPD are prevalent, and the patients who suffer from exacerbations frequently experience poor quality of life, accelerated decline in lung function, increased healthcare expenses, and increased morbidity and mortality. Studies testing patients’ sputum cultures have determined that approximately 50% of COPD exacerbations are attributed to bacterial lung infections.3 Bronchial colonisation by potentially pathogenic micro-organisms is related to the course of the illness, and is associated with airflow obstruction and a poor prognosis for many patients.4 Hunt et al.5 analysed how the pathogenic micro-organisms that persist within polymicrobial biofilms contribute to the severity of COPD exacerbations. They found that in animals exposed to smoke, infection by non-typeable H. influenzae led to airway neutrophilia, which is commonly seen in COPD. Neutrophilia is linked to airway obstruction and decline of forced expiratory volume in patients with COPD. Infection with non-typeable H. influenzae triggered the migration of neutrophils into the lungs, and increased the levels of active neutrophil enzymes, including myeloperoxidase, matrix metalloproteinase-2, and matrix metallopeptidase 9. The neutrophils release neutrophil elastase and produce neutrophil extracellular traps, which can degrade the extracellular matrix. Neutrophil extracellular traps are also responsible for increasing mucus viscosity, and thereby contribute to disease progression by complicating infections. Results of the study demonstrated that non-typeable H. influenzae infection led to alterations in lung morphology, obstruction of bronchioles, accumulation of mucus, and thickening of airway walls. In addition, infection led to significant airway resistance and a reduction in respiratory capacity. Overall, the infections caused by bacteria that reside within biofilms trigger host responses consistent with COPD exacerbations. Treating these biofilms may help alleviate airway inflammation, and potentially mitigate disease severity and slow the progression of COPD.5
Previously, the authors presented a detailed description of therapeutic approaches for COPD, with a focus on mucoregulators, bronchodilators, and anti-inflammatory drugs.6 In this article, the authors address approaches to inhibit and eradicate biofilms implicated in COPD pathogenesis.
MATERIALS AND METHODS
A meta-analysis of previously published studies was conducted. The search terms used included: “COPD exacerbation,” “anti-microbials,” “biofilms,” “quorum sensing,” “drug delivery,” etc. MEDLINE, Embase, related websites, and reference lists were searched from 2000–2023 to identify appropriate papers that addressed the objectives of this review. Publications were reviewed independently by four investigators. The investigators extracted the data and inspected each reference identified by the search and applied inclusion criteria. In cases where the same studies were reported in more than one publication, the study’s results were accounted for only once. The electronic search was followed by extensive hand searching, using reference lists from the identified articles. Publications written in English were reviewed exclusively. The search method used was designed to strengthen existing concepts, and to identify approaches for upcoming research studies.
BIOFILM FORMATION
Biofilms are dense micro-communities, often attached to inert surfaces that are encapsulated by secreted polymers.7 The development of a biofilm is a dynamic process.8 Initially, a planktonic microbe attaches itself to a surface; it then subsequently aggregates with other microbes in the formation of a biofilm.
The formation and survival of a biofilm lies in the ability of the bacteria to communicate. The bacterial cell–cell communication process, called quorum sensing (QS), involves the production, detection, and response to extracellular signalling molecules, autoinducers. QS controls genes that direct activities that are beneficial when performed by a population of bacteria acting in synchrony. Initially discovered in Vibrio fischeri, QS systems have been observed in Gram-positive and Gram-negative bacteria, as well as the fungus Candida albicans.9 The basic premise of QS is that the more cells are present, the more they can elicit a response from one another. Within the bacterial community, each individual cell produces a molecule termed an autoinducer, which exits the cell via passive diffusion in the absence of other autoinducers in the extracellular space. However, as the bacterial population grows, more autoinducers accumulate in the extracellular space, and the autoinducers begin to diffuse back down their concentration gradient, only this time, entering the cells. Once inside the cell, autoinducers bind to regulatory transcription factors in the cytoplasm or nucleus, inducing gene expression of the autoinducer synthase, which results in a positive feedback loop.10
The QS processes that occur in the bacteria that contribute to COPD exacerbation, are analogous to those seen in V. fischeri. In P. aeruginosa there are multiple known QS pathways, but two of them are nearly identical to the QS system in V. fischeri, LasI and RhlI. Similar to V. fischeri, both systems are activated by increased cell density.11 These genes encode the autoinducer synthase in P. aeruginosa, which allows for greater amplification of the signal.11 There is also evidence to indicate that QS is somewhat regulated by small RNAs, as well as other environmental factors.12 Additionally, biofilm formation can be inhibited when the secretion of matrix components ceases, a process that occurs through a tyrosine phosphatase that is controlled by the LasI system.13
There is a third and interconnected method of QS, which is based on quinolone signalling. In this pathway, the signalling molecule is 2-heptyl-3-hydroxy-4-quinolone, which is produced by multiple Pqs genes, one of which is PqsH. PqsH is under the control of the PqsH gene, and the molecule that detects the quinolone, PqsR, is encoded by the PqsR gene. The Las system induces the expression of these genes, while the Rhl system can suppress these genes.11
There is a fourth and newly discovered QS system that was discovered in P. aeruginosa, integrated QS. Although much about this system remains unknown, what is clear is that it is activated by phosphate depletion, and is controlled by Las like the quinolone system.14
The Las, Rhl, and quinolone systems function in tandem to create the functional elements of the biofilm that contribute to its stable structure and antibiotic resistant properties. One molecule that assists in keeping the biofilm intact is rhamnolipid, which creates channels between individual colonies to allow nutrients and other resources to travel amongst them. Pyoverdine is another molecule that is a product of QS, which assists in biofilm development by converting iron into stable forms so that it can be utilised by the cells within the biofilm. For maintenance of the biofilm structure, key elements are pyocyanin and pel polysaccharides. Pyocyanin causes the release of extracellular DNA into the environment, allowing it to bind the pyocyanin and pel polysaccharides, resulting in a more viscous biofilm matrix. Finally, lectin molecules are structural molecules that help hold the biofilm together and allow the biofilm to adhere to other surfaces.15
The autoinducer feedback loop mechanism of QS is present in nearly all gram-negative bacteria.16 In H. influenzae, another gram-negative microbe that is frequently found in patients who suffer from COPD exacerbation, there is an autoinducer QS system that is like the autoinducer system of P. aeruginosa, only with different autoinducers, genes, and regulatory transcription factors. An autoinducer, termed autoinducer-2 (AI-2), acts like the autoinducers in P. aeruginosa, activating S-ribosylhomocysteine lyase (LuxS), and acting as a positive feedback loop to produce an enhanced response.16 There is another QS system in H. influenzae that is unrelated to the autoinducer/LuxS system, which is the QS Escherichia coli B/C (QseB/C). QseC is a membrane-bound sensor that phosphorylates a QseB. Only when QseB is phosphorylated can it bind to DNA and alter transcription.17 While there is information on the signalling pathways involved in H. influenzae, how these directly lead to the formation of the biofilm is not as clear.18
Moraxella catarrhalis is yet another gram-negative bacterium that can colonise the lungs in an episode of COPD exacerbation. There is not much known about biofilms that consist of M. catarrhalis alone, but there is literature that explores biofilms that develop when there is coinfection of H. influenzae and M. catarrhalis. In such a system, the AI-2 method of QS is employed, but there are different theories that suggest how this occurs. One hypothesis is simply that AI-2 is a signal that is used by many species of bacteria since many contain LuxS. In certain cases, it appears that AI-2 can influence multi-species biofilms, which can be attributed to the presence of LuxS in more than one bacterial species. Another hypothesis is that M. catarrhalis does not have its own LuxS, so it cannot produce its own AI-2. However, it is nonetheless capable of responding to the AI-2 signals from the neighbouring H. influenzae.19
The final pathogen that is involved in COPD exacerbation is S. pneumoniae, which is a Gram-positive bacterium that can cause severe infection. Generally, one difference between Gram-positive and Gram-negative bacteria QS mechanisms is the type of molecule used for the autoinducer. While Gram-negative bacteria usually utilise small molecules as autoinducers, particularly lactones, Gram-positive bacteria will usually utilise peptides in their signalling, although the basic process of reactivating the autoinducer is the same in both systems.14
S. pneumoniae primarily uses two QS mechanisms: one is the autoinducer/LuxS process, and the second is the Com QS system. This system is focused on the exchange of DNA within the cells of the biofilm. This system is comprised of the ComCDE operon, where comC acts as the signalling molecule, comD acts as the receptor for comC, and comE regulates the transcriptional response. The exact role that both these systems play in the formation of the biofilm structure remains unknown.
CHRONIC OBSTRUCTIVE PULMONARY DISEASE EXACERBATION TREATMENT
The structure of a biofilm and the associated changes in gene expression can protect microbes from antibiotics.8 The treatment of infections in the context of chronic pulmonary disorders is limited by the biofilm mode of growth of pathogenic organisms. Compared with bacteria in their planktonic state, bacteria in biofilms can survive extremely high concentrations of antimicrobial compounds.20 A primary concern with antibiotic use in COPD is the emergence of antimicrobial resistance due to the frequent use of antimicrobials in this patient population. In addition, the COPD lung is susceptible to the formation of biofilms, thus enabling the development of antimicrobial resistance. Biofilms limit the infiltration of antibiotics and induce a heterogeneous community of bacteria that develop multidrug resistance (MDR).21 In many cases of infections involving biofilms, routinely used antibiotics, such as imipenem and colistin, reduce the biofilm, but are not successful in eradicating the biofilm completely.22 In respiratory infections involving biofilms, many practitioners resort to inhaled formulations of antimicrobial agents. Inhaled antimicrobials are beneficial because they allow for a higher concentrated dosage to reach the respiratory tract, thereby limiting systemic exposure of the antimicrobial compound. Some examples of inhaled antibiotics used to combat respiratory biofilms are ciprofloxacin, streptomycin, aztreonam, and colistin. Examples of inhaled antifungals include pentamidine and amphotericin B.23 While inhaled formulations limit systemic exposure of antibiotics, it is difficult to treat biofilms with single agent antimicrobials, because biofilms can survive 1,000-fold higher concentrations of antimicrobials compared with planktonic bacteria.24 The values of minimum inhibitory concentration required make antimicrobial agents less suitable for treating biofilms due to the increased risk of toxicity and side effects associated with the higher doses required.22 The MDR properties of biofilms warrant alternative approaches, such as enhancing activity of conventional antimicrobials synergistically, inhibiting biofilm formation, disrupting established biofilms, or by interrupting bacterial signalling pathways involved in biofilm formation.24 In addition, novel strategies in drug delivery have been utilised in recent years to combat biofilms implicated in respiratory infections.
ENHANCING ACTIVITY OF CONVENTIONAL ANTIMICROBIALS SYNERGISTICALLY
Several studies have demonstrated that dual antimicrobial therapy was more effective than monotherapy in eradicating biofilms in respiratory infections due to the tolerance of biofilms to antimicrobial agents. A study done by Gómez-Junyent et al.25 demonstrated that ceftolozane/tazobactam or meropenem used in conjunction with colistin was more effective in treating biofilms than with colistin alone. Lower bacterial counts of MDR P. aeruginosa strains were seen in the biofilm samples treated with combination therapy. Other approaches to combat biofilms include utilising non-conventional agents in conjunction with antibiotics. Results of a study done by Llamosí et al.26 revealed that when an antioxidant N-acetyl-L-cysteine (NAC) was used in combination with cefditoren, a cephalosporin antibiotic, NAC significantly enhanced inhibition and eradication of MDR S. pneumoniae biofilms. Similarly, enhanced anti-biofilm effects were seen when nitric oxide was utilised synergistically with antibiotics.27 While combination therapies are beneficial in eradicating biofilms, they pose an increased risk of side effects, allergies, toxicity, and increased risk of Clostridium difficile infections.28 To address the issue of toxicity, often seen with dual antimicrobial therapy, topical administration of these agents is recommended. Topical administration delivers high concentrations to the target site of infection with nearly undetectable serum concentrations thereby reducing the risk of systemic side effects. For example, when nebulised tobramycin was utilised to combat P. aeruginosa biofilms in cystic fibrosis human airway cells, serum concentrations remained <1 mg/L while it reaches levels up to 1,200 mg/L in the sputum.29
INHIBITING BIOFILM FORMATION AND DISRUPTING ESTABLISHED BIOFILMS
Recently there have been many advances in the utilisation of bacteriophage as an anti-biofilm agent. Bacteriophages can prevent biofilms from forming and can eradicate existing biofilms as well. Phages work by utilising enzymes to dissolve the outer matrix of the target biofilm, and once they penetrate the tough matrix, they continue to kill the bacterial cells embedded within the host biofilm.30 Antimicrobial peptides, also known as host defence peptides, are compounds found in the host defence systems, which offer multimodal antimicrobial effects through various complex mechanisms.31,32 A recent study highlighted the ability of antimicrobial peptides to interfere with production of extracellular polymeric substance, inhibit QS to prevent the formation of biofilms, and to kill cells adhered in biofilms.33 An example of an AMP that has been studied in respiratory infections includes amphibian AMP Esculentin (1–21). Luca et al.34 demonstrated that in mice with pulmonary infections and sepsis, those treated with amphibian AMP Esculentin (1–21) experienced prolonged survival due to the anti-biofilm effects of the AMP.34
QUORUM SENSING AND INTERRUPTING BACTERIAL SIGNALING PATHWAYS
In recent years, QS has become an attractive target to promote the dispersion of biofilms. Recent work has highlighted that acylated homoserine lactone lactonase AiiK, a synthetic anti-QS agent, was successful in preventing P. aeruginosa pyocyanin production, and thereby inhibited biofilm formation.35 Another attractive target is to block nucleotide signalling; nucleotides, such as cyclic guanosine monophosphate, serve as secondary messengers involved in gene regulation and transitioning of bacteria from planktonic to sessile state in the process of biofilm formation.36 Recent work has demonstrated that a decrease in cyclic guanosine monophosphate levels, through induction of E. coli YhjH cyclic guanosine monophosphate phosphodiesterase, induced the dispersal of bacteria embedded in P. aeruginosa biofilms in mice.37 Of note, while QS inhibitors can potentially prevent the maturation of biofilms, they do not affect the planktonic bacteria cells. As a consequence, QS inhibitors in concert with antibiotics may help control biofilm infections.9
ENHANCED DRUG DELIVERY
Biomaterial strategies describe the use of carriers to optimise the delivery of drugs to the target site of action. Recent work has highlighted the benefits of using nanoparticles. such as poly (lactide-co-glycolide) (PLGA), liposome, and chitosan, to enhance drug delivery when treating biofilms in the respiratory tract. These nanoparticles serve as promising advancements in the treatment of biofilms because they allow for controlled release of antimicrobials, and increase the penetration of the compound at the site of action. Furthermore, these drug delivery polymers shield the drug from degradation by the host and thereby enhance therapeutic effects of the agent.23,38,39 A study done by Lin et al.40 demonstrated that lysostaphin-encapsulated PLGA microparticles were successful in inhibiting the growth of methicillin-resistant Staphylococcus aureus, P. aeruginosa, and Klebsiella, and prevented the formation of biofilms. In those mice treated with the lysostaphin-encapsulated PLGA microparticles, the biomaterial allowed for enough of the lysozyme to be delivered to the lungs of the mice to eliminate methicillin-resistant S. aureus and improve survival rates.25,29 Bacteriophages and antimicrobial peptides have also demonstrated enhanced activity in the presence of biomaterials. In a study that evaluated the effects of an inhaled formulation of PLGA microparticles on the delivery of bacteriophage, results demonstrated that the PLGA microparticles loaded with bacteriophage significantly reduced bacterial load of P. aeruginosa and improved survival outcomes in mice infected with pneumonia.40 Table 1 summarises several biofilm inhibition strategies.
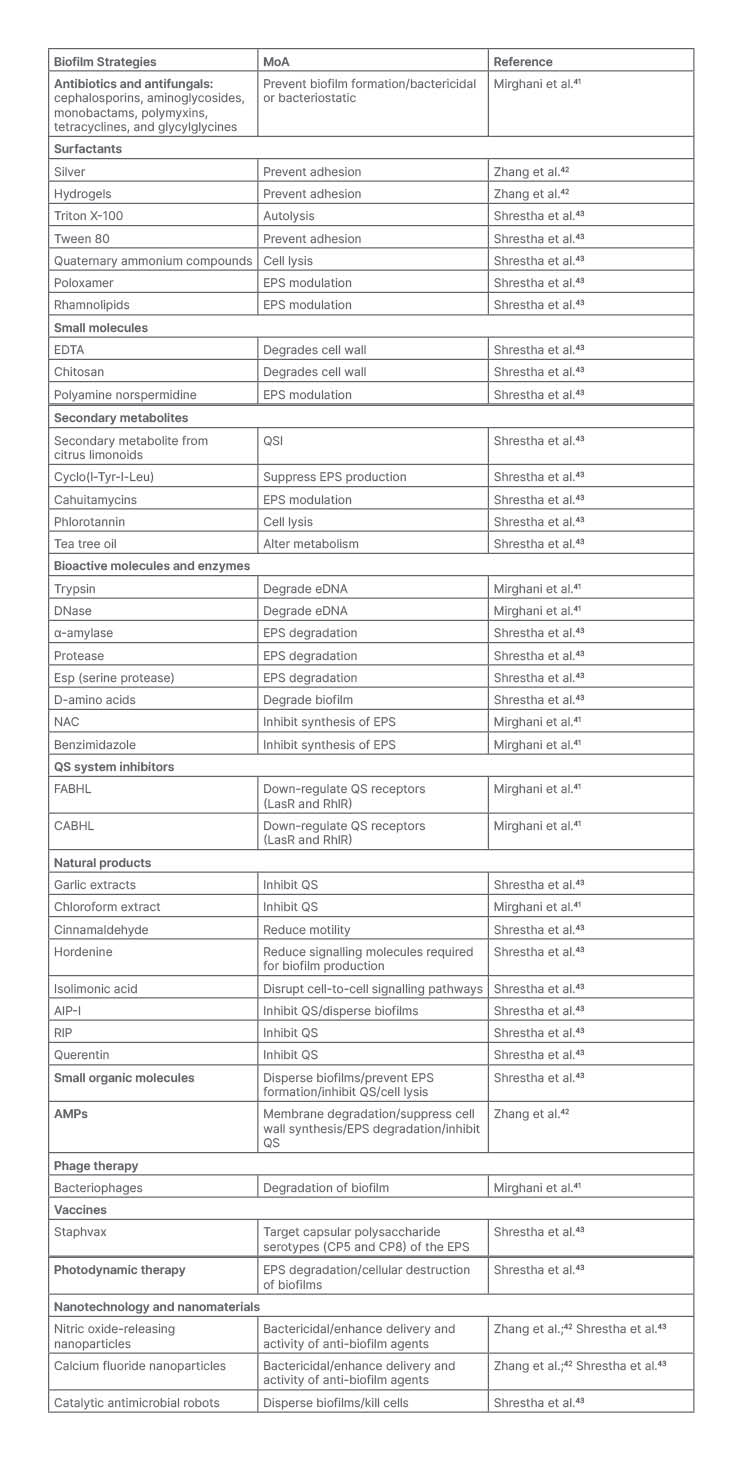
Table 1: Biofilm inhibition strategies.
AIP-I: autoinducing peptide Type I; AMP: antimicrobial peptide; CABHL: N-(4-[4-chlororoanilno]-butanoyl)-L-homoserine lactone; DNase: deoxyribonuclease; eDNA: environmental DNA; EDTA: ethylenediaminetetraacetic acid; EPS: extracellular polymeric substance; FABHL: N-(4-[4-fluoroanilno]-butanoyl)-L-homoserine lactone; NAC: N-acetylcysteine; QS: quorum sensing; QSI: quorum sensing inhibitor; RIP: RNAIII-inhibiting peptide.
CONCLUSIONS
World-wide, COPD poses significant health and economic challenges. According to the World Health Organization (WHO), 3.23 million people died from COPD in 2019.44 Direct medical costs attributed to exacerbation approximates 83% of the total healthcare costs for COPD.45 Although much progress has been made in the treatment of COPD, advancements are needed in inhibiting and eradicating biofilms implicated in COPD exacerbation.
This review summarised the current approaches used to combat biofilm-mediated COPD exacerbation. The authors demonstrated that microbial SQ provides for attractive therapeutic targets. That said, more work is needed on strategies designed to modulate microbial behaviours. Presumably, therapies that affect microbial behaviour will not be prone to resistance as are traditional antibiotics and will thus yield promising results.