Abstract
Conventional imaging modalities, the X-ray and CT, are essential diagnostic tools in respiratory medicine, providing qualitative information regarding the pattern and extent of pulmonary pathology. Neither provide information regarding the functional impact of pulmonary diseases and additional investigations are required to assess this. Hyperpolarised xenon-129 MRI (HPX-MRI) is a non-invasive, ionising-free imaging modality that can provide functional measurements of ventilation and diffusion within the lung. The MRI-based technique involves the subject breathing in an inert gas that has been hyperpolarised to make it visible using MR spectroscopy. The gas mirrors the flow of oxygen through the bronchial tree, across the alveolar membrane, and into the pulmonary capillaries, and thus disruptions in the passage of gas due to ventilation and diffusion abnormalities can be visualised and quantified. Functional measurements of ventilation and gas exchange within regional areas of the lung can be obtained. HPX-MRI has the potential to detect early lung disease not yet evident using standard investigations and has been shown to be a sensitive modality to assess treatment responses. The role of HPX-MRI in respiratory medicine has the potential to be wide-reaching, but to date it remains largely a research tool. This review article summarises the current and possible future clinical applications of HPX-MRI in the investigation and management of lung diseases, pitched at a level comprehensible and relevant to the respiratory-focused clinicians.
Key Points
1. Hyperpolarised xenon-129 MRI (HPX-MRI) is a functional imaging technique that provides global and regional measurements of ventilation and gas exchange and can assist in the assessment and management of respiratory diseases.2. An overview of the technology and clinical applications of HPX-MRI, aimed at respiratory-focused clinicians.
3. HPX-MRI is a functional imaging modality that is safe, quantitative, and reproducible. Clinical studies have highlighted its role in phenotyping respiratory pathology, early-stage disease detection, assessing progression, and therapeutic response to treatment.
INTRODUCTION
The advent of CT has enabled the assessment of different tissue densities of internal organs in ‘slices’, and thereby transformed the diagnosis and management of many respiratory diseases.1,2 Although CT provides valuable spatially resolved information about lung structure and pathology, correlating radiological findings with functional investigations is necessary to understand the physiological impact of a disease. MRI is capable of producing detailed images of soft tissue structures; however, until recently, its utility for investigating pulmonary conditions has been limited. The development of hyperpolarised gas MRI has enabled functional imaging of the airways and alveoli and provides a reliable measure of gas exchange, improving our understanding of respiratory pathology.3,4 Hyperpolarised gas MRI can identify regional areas of abnormality and directly measure the physiological impact of disease locally and for the whole lung. This review summarises the current and potential applications of hyperpolarised xenon-129 MRI (HPX-MRI) in the investigation and management of respiratory diseases.
Conventional MRI relies on the signal generated from exciting protons to generate images. As the lung parenchyma has a high air content and low proton density, the signal generated from the lung is limited and precludes adequate structural assessment.5 More recently, there have been some advancements in MRI resulting in enhanced visualisation of lung structure using techniques such as ultra-short echo-time and zero echo-time imaging. These approaches acquire data from the rapidly decaying signal from protons in the lungs, providing improved visualisation of structure in comparison to conventional MRI, but still does not provide visualisation of lung function.6,7
For many lung diseases, high-resolution CT imaging provides a diagnosis, informs the need for treatment, and enables longitudinal monitoring of disease.8 However, traditional imaging does not assess the functional impact of diseases and may poorly differentiate incidental findings from disease in its early stage when it may be reversible or more readily treatable.9 Current pulmonary function testing in clinical practice provides a useful ‘global’ assessment of lung function but lacks the ability to localise areas of lung abnormality. Given the heterogeneity of many pulmonary diseases and the way they progress, assessing regional function may provide important prognostic information. Furthermore, traditional pulmonary function testing is reliant on patient effort and technique and is associated with a degree of inherent variability (up to 15% for gas transfer), and consequently may not always provide reliable measurements.10-12 These limitations highlight the advantage of novel and more sensitive diagnostic techniques that can both detect and assess functional components of lung disease.
Current functional imaging techniques used in clinical practice are planar ventilation/perfusion (V/Q) scintigraphy and single photon emission CT (SPECT) V/Q. These display the distribution of radiolabelled gas and macroaggregates of albumin during the ventilation (V) and perfusion (Q) phases, respectively.13,14 These planar images provide limited spatial information, which although improved using SPECT, has significantly lower spatial resolution than hyperpolarised gas MRI.15,16 SPECT V/Q enables disease quantification but involves ionising radiation, limiting its use for repeated scanning in patients with chronic disease.14,17
The development of hyperpolarised noble gases, helium-3 (3He) and xenon- 129 (129Xe), as inhaled contrast agents in pulmonary MRI started in the 1990s.18-21 The inhalation of hyperpolarised xenon- 129 enables direct visualisation of functioning lung structure as it flows and fills the airways and alveoli, mirroring the passage of oxygen.22 Abnormalities in ventilation due to airway narrowing or bullae formation can be qualitatively and quantitatively measured.15 Defects in the transfer of gas across the alveolar capillary can also be assessed, providing a sensitive measurement of the membrane and pulmonary vasculature integrity.3
HPX-MRI is a state-of-the-art non-invasive, ionising-radiation-free functional imaging modality that is reproducible, quantitative, safe, and involves minimal patient effort.3,4 The potential role of this modality in respiratory medicine is wide-reaching but it remains, to date, largely a research tool. Clinical studies, however, indicate a valuable role in enhanced phenotyping, early-stage disease detection, therapeutic guidance, and improving our understanding of disease mechanisms.23
HYPERPOLARISED XENON-129 MRI
Conventional MRI relies upon the signal provided by protons primarily attached to water and fat molecules. Other atoms visible in an MRI scanner include the inert atmospheric gas, xenon-129, phosphorus-31, and sodium-23/carbon-13, which are present at very low natural abundance, and so detecting signals from these atoms in vivo is challenging.24 However, using concentrated xenon-129 gas and a process known as ‘hyperpolarisation’, it is possible to transiently boost its signal by approximately 5–6 orders of magnitude for a limited time.3,24,25 This, in turn, provides enough signal to directly image the spatial distribution and movement of inhaled xenon-129 gas in vivo. Hyperpolarisation is achieved when a gas mixture containing xenon-129 is fed into an optical cell containing Rubidium (Rb) vapour and a laser polarises the Rb atoms. The resulting Rb-129Xe interaction transfers the electron spin polarisation to the xenon-129 nuclei.3 This process is called spin exchange optical pumping.
To image xenon-129, a radiofrequency coil (fitted around the chest) and a broadband radiofrequency amplifier and software (for tuning to the resonance frequency of xenon-129) are required for signal detection.4 These devices are not commonplace in most MRI units and require additional funding to purchase. The scan process involves the patient inhaling up to 1L of xenon-129 from a gas delivery bag and holding their breath for around 10–15 seconds whilst lying in the MRI scanner. Xenon-129 has been evaluated for its safety and tolerability, and major adverse effects have not been reported. Mild side effects are recognised, but are transient and include dizziness, paraesthesia, and euphoria.26-28
The process of hyperpolarisation therefore enables the signal from xenon-129 to be detected within the lungs after it is inhaled by the subject. Airway abnormalities causing ventilation defects, such as bronchial wall narrowing due to asthma, appear as regional signal defects and can be quantified using a gas distribution cluster-map. In this form of static ventilation imaging, measurement of ventilation deficits is represented by the ventilation defect percentage (VDP), defined as the fraction of unventilated lung. This is calculated from the ventilation defect volume divided by thoracic cavity volume, expressed as a percentage (Figure 1A).29,30
Delays in the diffusion of gas across the alveolar-capillary epithelium into red blood cells (RBC) due to structural abnormalities at this interface or the pulmonary vasculature, can be quantified and assessed using ‘dissolved-phase’ imaging. After inhalation, xenon-129 diffuses from the alveoli (gas-phase) into the alveolar capillary membrane. About 2% of the inhaled xenon-129 then diffuses into the capillary blood stream, where it transiently binds with haemoglobin in RBCs (dissolved-phase).3,31-33 Due to an MRI phenomenon known as ‘chemical shift’, xenon-129 exhibits different resonance frequencies within the three different compartments. Following detection and reconstruction of the MR data, it is possible to assess the ratio of xenon-129 within gas phase, tissue barrier and plasma (TP), and RBC components (Figures 1B and 2A).24,34,35 Typically, 1–2% of the xenon-129 signal originates from xenon-129 dissolved in the lung tissue, and is not exclusively from xenon-129 bound to haemoglobin.
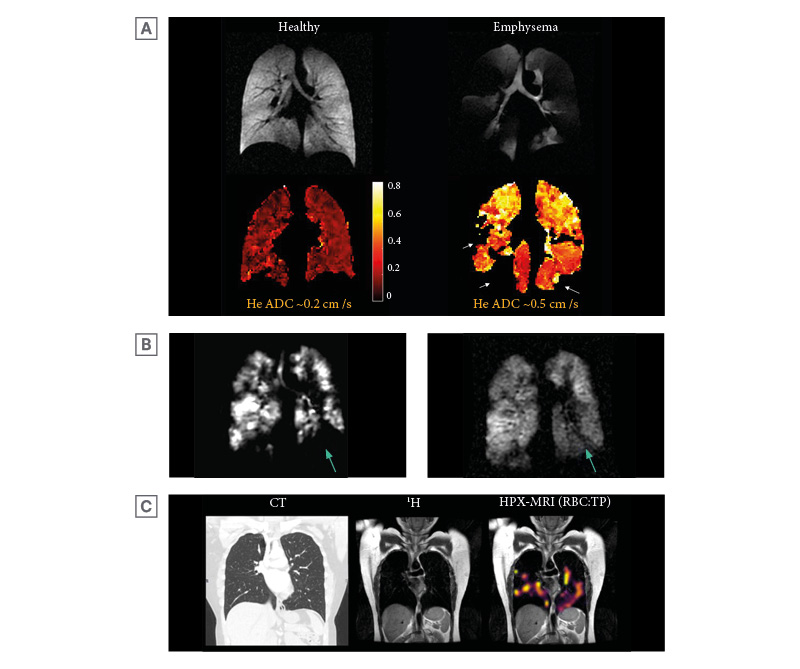
Figure 1: Example images of hyperpolarised xenon-129 MRI.
A) HPX-MRI ventilation imaging showing xenon-129 gas distribution (yellow to purple) within the tracheobronchial tree and lung fields in a young healthy control. B) Examples of the gas, TP, and RBC phases (not corrected for coil inhomogeneity) reconstructed into HPX-MRI ratio maps in a healthy volunteer. The gas phase demonstrated distribution of xenon-129 gas within the airways with no evidence of ventilation defects, indicative of normal airway calibre. The TP phase similarly demonstrated distribution of xenon-129 as in the gas phase, indicating normal gas transfer from the airways into the interstitial membrane and plasma. The RBC phase also demonstrated distribution of xenon-129 similar to the TP phase, demonstrating normal gas transfer into the red blood cells from the interstitial membrane and plasma. C) HPX-MRI ventilation images depicting ventilation defects (arrows), which improved following bronchodilator administration. There are other areas of persistent ventilation defects that had not resolved following bronchodilator administration. Original image provided by POLARIS group, University of Sheffield.
HPX-MRI: hyperpolarised xenon-129 magnetic resonance imaging; RBC: red blood cell; TP: tissue barrier and plasma.
It is also possible to assess the movement of xenon-129 gas within the alveoli using ‘diffusion-weighted imaging’, and from this, obtain information about the integrity of the alveoli.3 Gas movement (referred to here as ‘diffusion’) is mainly limited by the proximity of the surrounding tissue. Therefore, in diseases where there is destruction of the alveolar architecture (e.g. emphysema), gas can move more freely within the remaining enlarged alveolar spaces (Figure 2B). This is represented by apparent diffusion coefficient (ADC) that measures the Brownian motion of xenon-129 within the alveoli, with increased values denoting greater movement of gas due to reduced alveolar integrity.3
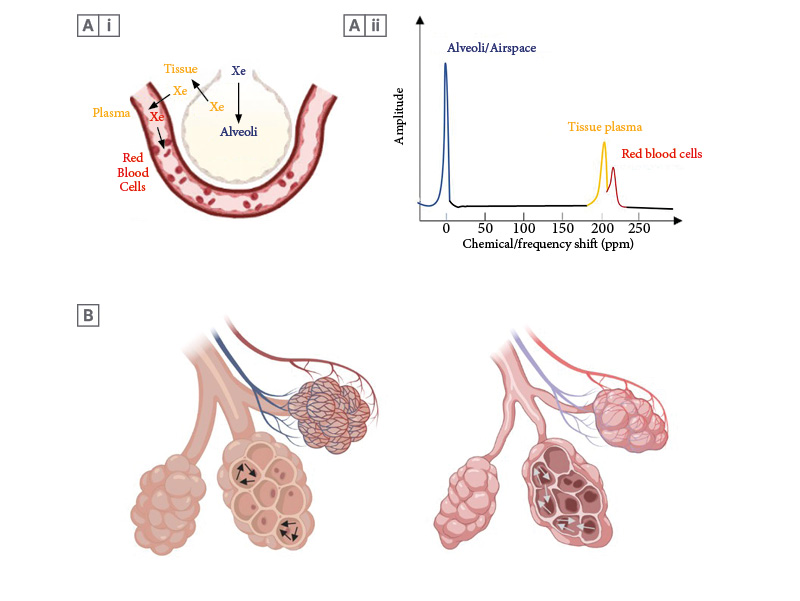
Figure 2: Illustrative images showing the movement of xenon gas within the alveolar capillary unit.
A(i) Similar to oxygen, xenon-129 moves into the alveoli during the ventilation phase, before diffusing into the tissue/alveolar membrane and plasma (blue to yellow). Xenon-129 then binds to the red blood cells (yellow to red). (Created with Biorender.com). A(ii) The chemical/frequency shift represents a change in the resonance frequency of xenon-129, due to alterations in the chemical environment within each compartment. When xenon-129 atoms move between compartments, the xenon-129 signal frequency changes instantaneously, occurring simultaneously with the physical movement of xenon atoms. This figure depicts the distinct frequency shifts of xenon-129 on the xenon-129 spectrum. The colour of each frequency shift in each compartment corresponds with the colour of xenon-129 in each compartment in Panel Figure A(i). B) Schematic description of gas flow (arrows) within the alveoli, with more free-moving gas in emphysematous alveoli due to destruction of alveolar tissue contributing to raised ADC in COPD. (Created with Biorender.com).
ADC: apparent diffusion coefficient; COPD: chronic obstructive pulmonary disease; ppm: parts per million.
CLINICAL APPLICATIONS
Obstructive Airway Diseases Asthma
Assessment of regional ventilation defects, disease severity and treatment response
Asthma is a chronic disease characterised by airway hyper-responsiveness and inflammation, resulting in increased airways resistance.36 The severity and distribution of the disease can be both visualised and quantified by assessing the pattern of xenon-129 within the airspaces after inhalation using ventilation maps. Typically, a heterogeneous distribution of gas is observed and ventilation defects (indicated by raised VDP) have been reported, even in cases of well-controlled asthma (Figure 1C).29,30,37-40 Heterogeneity is normally represented by the coefficient of variation, which is calculated as the ratio of the standard deviation to the mean of the xenon-129 signal intensity. In keeping with the typically reversible nature of the disease, Svenningsen et al.29 and Safavi et al.37 found significant improvement in the extent of ventilation defects, alongside a reduction in the coefficient of variation on ventilation maps, following bronchodilator treatment (Figure 1C).
VDP has been found to correlate well with forced expiratory volume in 1 second (FEV1), FEV1/forced vital capacity (FVC) ratio, forced expiratory flow between 25% and 75% of vital capacity, fractional exhaled nitric oxide, and lung clearance index (LCI).30,37-42 LCI is a non-imaging lung function measurement technique commonly used in children unable to undertake spirometry. LCI assesses small airway inhomogeneity by measuring the ventilation distribution of a tracer gas over cycles of tidal breathing, and has been found to be more sensitive at detecting ventilation abnormalities than FEV1.43,44 Following bronchodilator challenge, Safavi et al.37 found an improvement in VDP, but not in LCI, in children with asthma, suggesting the potential capability of HPX-MRI to detect more subtle ventilation inhomogeneity than LCI and greater sensitivity in assessing response to treatment. The VDP may also provide a more accurate measure of disease severity, with Lin et al.42 showing that higher VDP scores correlated well with clinical severity in children, assessed by number of healthcare attendances and use of corticosteroids. Given the sensitivity of HPX-MRI in identifying ventilation defects, it may also provide a more sensitive method of assessing and monitoring treatment response in comparison to conventional lung function tests (LFT). McIntosh et al.45 and Kooner et al.46 reviewed the impact of the novel biologic treatment, benralizumab, which showed improvement in VDP, although not in FEV1 or LCI, as early as 14-days post-treatment.
HPX-MRI also has a potential role in guiding bronchial thermoplasty. Ventilation defects have been shown to correspond to airway narrowing, and Svenningsen et al.47 undertook HPX-MRI on patients with asthma, pre-procedure, to identify the most severely affected airways.47 Investigators found that this image-targeted approach reduced the number of thermoplasty treatments required compared to patients enrolled in the standard non-image guided arm.47,48
Chronic Obstructive Pulmonary Disease
Assessment of regional ventilation defects, integrity of alveolar tissue and lung function
Chronic obstructive pulmonary disease (COPD) is characterised by persistent respiratory symptoms resulting from airflow obstruction secondary to chronic inflammation, and a variable reduction in the integrity of the lung parenchyma due to the presence of emphysema.49 HPX-MRI can be used to quantify ventilation defects through the VDP and provide a measure of the quality of alveolar tissue via diffusion (ADC) measurements (Figure 2B and 3A). In COPD, the VDP and ADC are raised, with regional measurements typically highest in the upper lobes where emphysema is most prevalent.50-55 The ADC correlates with measures of airflow obstruction on LFT, diffusing capacity of the lungs for carbon monoxide (DLco) transfer factor, and quantitative emphysema scores on CT.50,51,53-58 Similar to asthma, the VDP correlates well with FEV1 and FEV1/FVC ratio.
HPX-MRI enables the assessment of gas exchange at the alveolar-capillary membrane, providing an additional measure of functional deficit in COPD. Calculating the ratio of xenon-129 in the gas phase (i.e. within the alveoli), TP (representing the alveolar capillary membrane and plasma), and RBC from dissolved-phase imaging can provide sensitive gas transfer measurements. In COPD with emphysema, reductions are typically seen in all ratios: the TP:Gas (a measure of gas diffusion across the interstitial tissue providing information on tissue density), RBC:Gas (the HPX-MRI counterpart of DLCO, and a measure of gas transfer from alveoli into the RBCs), and RBC:TP (a measure of gas exchange efficiency from interstitial tissue to blood).55,59
HPX-MRI may play a useful role in the early diagnosis of COPD when LFT parameters remain within normal range. Functional alveolar wall thickness can be computed from xenon-129 uptake, and has been shown to be increased in ‘healthy’ smokers with normal lung function compared with healthy non-smokers, thereby detecting the early-stage changes associated with COPD.57,60-62 Using an innovative 3D alveolar gas-exchange model extrapolated from HPX-MRI dissolved-phase imaging data, the functional volume of pulmonary tissue, capillaries, and veins can be measured.63 Unsurprisingly, this is diminished in COPD compared to healthy controls, but interestingly, significant reductions in the functional volume of pulmonary tissue were also noted in patients with only very minimal emphysematous changes on CT. This suggests a potential additional method of assessing asymptomatic early-stage disease.
A further clinical application of HPX-MRI is the potential detection of collateral ventilation in severe COPD. Collateral ventilation refers to the direct flow of air between alveolar structures, bypassing the normal airway route.64 In severe emphysema, where lung volume reduction treatment is being considered, establishing the presence of collateral ventilation between lung lobes is essential to determining the likelihood of procedural success. Traditionally, this is assessed by two modalities: quantitative CT analysis of lung fissure integrity, and through endobronchial catheter occlusion of the lobar airway using the Chartis system® (PulmonX Inc., Redwood City, California, USA).65 Using the Chartis system®, if there is no collateral ventilation, the occluded airway results in lobar lung collapse following the one-way expiration of air. However, in the presence of collateral ventilation, the lung lobe does not deflate due to the flow of air from adjacent lobar structures. Whilst this method is sensitive, it is invasive, time consuming, and requires technical expertise available only in a limited number of specialist centres. Advances in dynamic HPX-MRI ventilation imaging may provide a more convenient and non-invasive alternative, enabling the measurement and visualisation of collateral ventilation in patients with COPD. This has been evaluated in studies by Marshall et al.66,67 and Chen et al.68 who used 3He-MRI and HPX-MRI, respectively, to assess the presence of collateral ventilation, demonstrated by delayed filling of gas in non-ventilated regions (Figure 3B).
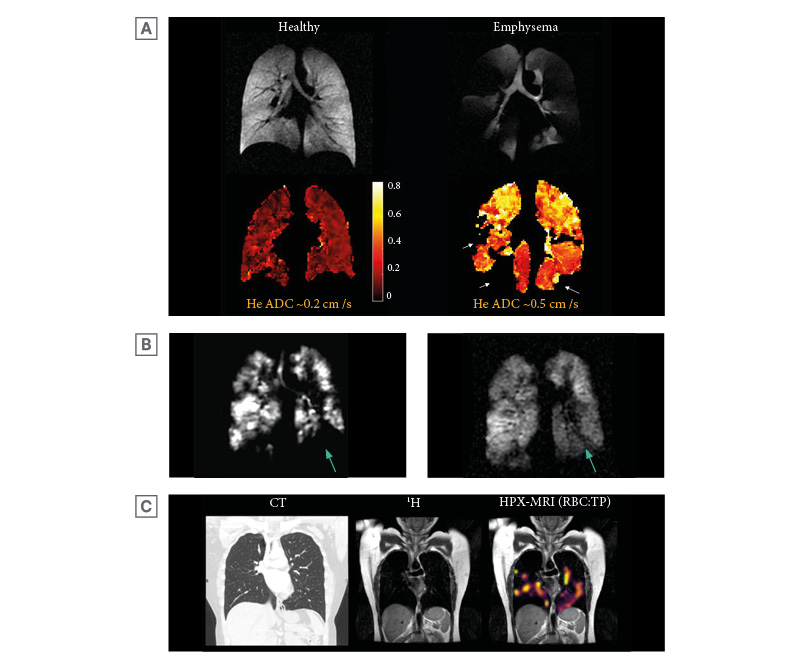
Figure 3: Example images of hyperpolarised helium-3 and xenon-129 MRI.
A) An example of helium-3 MRI ADC maps (HPX-MRI produces similar ADC maps) with corresponding ventilation images in a healthy volunteer and a patient with emphysematous lungs, highlighting higher ADC (bright yellow) in areas of abnormal emphysematous lungs. The patchy voids (arrows) in the ADC map of the patient with emphysematous lungs signify areas of ventilation defect where ADC cannot be quantified. The whole lung mean ADC of approximately 0.5 cm2/s was higher in emphysematous lungs than in healthy lungs (approximately 0.2 cm2/s). Original image provided by POLARIS group, University of Sheffield. B) HPX-MRI images illustrating the dynamic filling of ventilated defects (arrow) in a patient, suggesting the presence of collateral ventilation. C) Chest CT, proton (1H), and RBC:TP HPX-MRI images illustrating the heterogenous pattern of xenon-129 signal and reduced RBC:TP ratio in a patient not hospitalised for COVID-19 infection with unremarkable lung parenchyma on chest CT, months after the initial infection.
ADC: apparent diffusion coefficient; HPX-MRI: hyperpolarised xenon-129 magnetic resonance imaging; RBC: red blood cell; TP: tissue barrier and plasma.
Idiopathic Pulmonary Fibrosis
Assessment of septal wall thickness, lung function, disease severity and prognosis
Idiopathic pulmonary fibrosis (IPF) is a progressive fibroproliferative disease characterised by the progressive accumulation of scar tissue that gradually replaces the normal lung parenchyma.69 Established fibrosis is evident on CT, and disease progression is traditionally assessed through worsening CT findings and a fall in the LFT parameters, such as FVC and DLCO. The role of HPX-MRI has been investigated in several research studies, and findings suggest it may be a more sensitive way of evaluating disease progression and response to treatment.
Unsurprisingly, studies using HPX-MRI dissolved-phase imaging to measure the RBC:TP and RBC:Gas have found the ratios to be consistently lower than in controls, signifying reduced gas transfer due to thickening and fibrosis of the interstitium.70-81 These dissolved-phase parameters strongly correlated with measurements of septal wall thickness and DLCO. However, RBC:TP has the advantage over DLCO in that it is more reproducible (and therefore more accurate) and can directly measure the function of alveolar-capillary membrane regionally.82 It can be measured continuously after inhalation of xenon-129, independent of the patient’s effort. In IPF, the pattern of HPX-MRI abnormalities is typically most marked in the basal and peripheral lung regions, consistent with the distribution of disease.74,78
Studies comparing histology and CT findings have shown that early fibrotic changes identified histologically may not be evident on CT.83,84 Through the ability of HPX-MRI to probe the lung parenchyma functionally, regional abnormalities in gas transfer can be identified that may be below the resolution of CT.72,85 Hahn et al.72 reported that patients with IPF who have progressive disease demonstrated a reduction in the RBC:TP in normal appearing lung, in comparison to those who did not progress over the study period.72 Comparing visually detected and semi-quantitative assessed lung fibrosis with HPX-MRI parameters, no significant correlation was seen, suggesting that functional HPX-MRI changes may predate radiological fibrosis on CT.77,79
Studies evaluating HPX-MRI dissolved-phase imaging in IPF have found that the uptake of xenon-129 into the interstitial tissue (represented by TP compartment) is raised, demonstrated by an elevated TP:Gas ratio.70,74,76,77,79,81 This indicates that there is a larger tissue volume relative to the alveolar gas volume for xenon-129 to dissolve. The apparent ratio increase can be due to fibrotic tissue formation, as well as a reduction in lung compliance, which reduces the ventilation volume and thereby increases the TP:Gas ratio. Interestingly, this finding has been identified by Qing et al.79 even in patients with normal or mildly impaired pulmonary function tests and radiologically early disease, suggesting that HPX-MRI may pick up early fibrosis, or possibly areas of active fibrogenesis, which theoretically may have a reversible component.79 HPX-MRI may also provide important prognostic information. Rankine et al.80 found that the presence of both abnormal TP and RBC phases inferred an increased risk of mortality and/or the need for lung transplantation.
Long COVID/Post-COVID-19 Syndrome
Assessment of lung function
Post-COVID-19 syndrome, more commonly known as long COVID, has been defined by the National Institute for Health and Care Excellence (NICE) guideline as ‘signs and symptoms that develop during or following an infection consistent with COVID-19, which continue for more than 12 weeks and are not explained by an alternative diagnosis’.86 Breathlessness is a particularly common symptom, and conventional investigations are often unremarkable.
Several studies have examined the role of HPX-MRI to investigate breathlessness in previously hospitalised patients with COVID-19. Li et al.87 evaluated patients at baseline and at 1-month follow-up after hospital discharge with COVID-19 pneumonia and identified the presence of ventilation defects on HPX-MRI. These abnormalities were co-located within areas of residual ground glass opacification related to the primary pneumonic process. The ground glass changes on chest CT were also associated with reduced gas transfer and functional septal wall thickening, although interestingly, reduced RBC:TP ratio and raised VDP were also seen within normal areas of lung on CT. This likely indicates the persistence of microstructural damage following radiological resolution. Furthermore, Grist et al.88 showed impaired gas transfer measured by the RBC:TP ratio in 12 patients post-COVID-19, despite a normal or near-normal chest CT 3–6 months following hospital discharge. Matheson et al.89 reported similar findings with significantly reduced RBC:TP ratios 6–12 months after infection in 12 patients post-hospitalisation.The presence of persisting functional lung abnormalities following COVID-19 pneumonia severe enough to warrant hospitalisation is not necessarily surprising. Patients not hospitalised for COVID-19 rarely have evidence of a prior pneumonic illness on their chest CT scans, although breathlessness in this group is a common finding. Interestingly, in a small pilot study by Grist et al.88 seven out of 11 non-hospitalised patients from the first wave of the pandemic were found to have significant reductions in RBC:TP ratio and DLCO 3–15 months after their initial infection.
Although the participant numbers within these studies were small and the association with symptoms unclear, it is of note that many of the participants had their initial infection over 6 months previously, indicating that the abnormalities seen cannot be explained by a transient viral lung injury (Figure 3C).88,89 The pathophysiology underlying these HPX-MRI abnormalities is also unknown, although, the low RBC:TP ratio suggests that the defect lies at the alveolar-capillary membrane due to either alveolar membrane damage and/or disruption of pulmonary blood flow. A larger multicentre research trial is currently underway to further assess the impact of COVID-19 on lung function using HPX-MRI.90
Other lung conditions
The role of HPX-MRI has also been evaluated in a range of other diseases, including cystic fibrosis (CF), lymphangioleiomyomatosis, and pulmonary hypertension (PH). In each condition, the potential main advantage of HPX-MRI over conventional investigations lies in its ability to detect early abnormalities and identify subtle changes in disease status, both in terms of treatment response and disease progression.
In CF, for example, ventilation abnormalities can be observed through VDP measurements in the absence of lung function changes.91-104 Following antibiotics during CF exacerbation, Rayment et al.105 found a larger improvement in the VDP than either the FEV1 or LCI, suggesting superior sensitivity of the VDP over other measurements. In lymphangioleiomyomatosis, early cystic lung changes have been identified and can be functionally assessed through VDP and ADC measurements when spirometry values are preserved.96,106 In the setting of PH, which is formally diagnosed via right heart catheterisation, the role of HPX-MRI has been evaluated as a non-invasive alternative.107-109 Comparing HPX-MRI parameters to COPD, IPF, and heart failure, PH exhibited unique characteristics with focal reductions in gas transfer (RBC:TP).107 Investigators found that in the absence of lung disease, HPX-MRI had good diagnostic accuracy when compared to right heart catheterisation in pre-capillary disease, although, further work looking at its application in a larger cohort and different subtypes of PH is required.108 HPX-MRI has also been studied in paediatric populations. In this setting, the modality has the advantage over conventional lung function tests due to the ease of performing the technique, as only a single breath hold is required, rather than multiple breathing manoeuvres. Furthermore, HPX-MRI measurements have been found to be highly reproducible, even between centres, making it appealing as a longitudinal tool.101 HPX-MRI has been trialled as an alternative method to detect ventilation abnormalities in children who were unable to perform spirometry following bone marrow and stem cell transplant.95,96,110 In bronchopulmonary dysplasia, a congenital condition characterised by diminished lung tissue due to immature alveoli formation, HPX- MRI has been utilised to quantify the extent of functional impairment through the reductions in the TP:Gas ratio.95
CONCLUSION
This review has summarised the versatility and potential clinical applications of HPX-MRI in the diagnosis and phenotyping of pulmonary diseases. Studies have shown that it can provide reliable measurements of disease severity, assess progression, and monitor treatment response more sensitively than conventional methods. HPX-MRI has the advantage over traditional lung function modalities by providing regional and spatial functional measurements and can detect evidence of preclinical disease. Its utility in pre-procedure assessments, such as lung volume reduction procedures, may negate the need for invasive tests. However, to date, most HPX-MRI studies have involved relatively small patient cohorts, and as a modality, it remains within the research realm. With technological advances, including improved transportability of hyper-polarised xenon-129 gas, larger multi-centre site studies are needed to further evaluate its role in different disease settings and assist in its translation into clinical practice.