Abstract
Paediatric cancer treatment has advanced significantly over the last half century to a point where >80% of all childhood cancer cases survive >5 years from diagnosis. However, childhood cancer treatments cause a wide range of long-term adverse effects including endocrine dysfunctions, impaired physical function, and a markedly increased risk of developing metabolic and cardiovascular complications. Emerging evidence suggests that treatment-related muscle toxicities may play a key role in the development of such late effects, but limited research has been performed towards elucidating this phenomenon and therapeutic countermeasures are scarcely available in clinical practice. Here, we review the current literature describing the physiological manifestations of treatment-induced muscular toxicities in paediatric oncology and discuss the use of structured exercise as a targeted countermeasure.
INTRODUCTION
Paediatric oncology therapy is a major medical success story largely owing to progressive, co-ordinated development and experimental testing of risk-based therapies,1 leading to impressive improvements in 5-year relative survival rate which is currently >80%.2 However, curative childhood cancer treatment is associated with a wide range of short and long-term complications constituting a major health concern in post-treatment survivors, including endocrine dysfunction,3 functional capacity deficits,4-8 and a markedly increased risk of metabolic and cardiovascular diseases.3,9-13
Accordingly, substantial attention within childhood cancer survivorship is directed towards elucidating the clinical implications and underlying biological mechanisms responsible for serious late effects following curative treatments. Strong evidence has outlined the negative consequences of treatment-related endocrine deficiencies, cardiomyopathy, and endothelial dysfunction,3,11,12,14-18 whereas limited attention has been directed towards the potential role of treatment toxicities in skeletal muscle, a known regulator of overall physical capacity and the foundation for functional independence. Reports in long-term survivors of childhood cancer indicate that patients are subjected to muscle toxicities which may manifest as poor physical function many years after cessation of treatment.4-7 However, there is limited evidence available describing muscular toxicities from childhood anti-cancer therapy, including the role of treatment-induced muscle dysfunction in the aetiology of late-occurring metabolic disorders and their mechanisms of action.
Against this background, we present a conceptual outline of childhood cancer treatment with regard to skeletal muscular toxicities and their potential long-term implications for metabolic deficiencies and complications. We review the most common treatment modalities in modern paediatric oncology with a specific view towards their possible adverse impact on muscular biology and regulation, and discuss the emerging application of exercise training in paediatric oncology as a pleotropic strategy to minimise and/or reverse the treatment-induced muscular dysfunction.
CHILDHOOD CANCER MANAGEMENT
Multi-modality treatment approaches using multi-agent chemotherapy in combination with surgery and radiotherapy have led to improved survival rates and subsequently increased focus on treatment-related adverse late effects. Studies have demonstrated that ≤90% of childhood cancer survivors suffer from a chronic health condition by the age of 45.19-20 Thus, while improvement in overall survival has been marginal over the last decade, progress in paediatric oncology has largely involved the adoption of a risk-adapted therapeutic approach. Specifically, the identification of clinical and biological prognostic factors has provided the ability to stratify patients and modify treatments accordingly, allowing treatment to be intensified in the high-risk patients, while therapy for low-risk patients can be decreased to minimise toxicities and the risk of adverse late effects without compromising survival. The concept of improved childhood cancer outcomes is no longer confined to better overall survival, but also focussed on minimisation of the prevalence and severity of short and long-term treatment-related complications.
Several cross-sectional studies have shown that survivors of childhood cancer present with low muscle strength many years after treatment,4-7 which can translate into limitations in physical performance4-6 and low quality of life.21 While late-occurring muscle dysfunction has long been recognised as a clinical challenge in paediatric oncology, it has only recently been suggested that this may constitute an intrinsic feature associated with early onset and/or high incidence of metabolic complications in childhood cancer survivors.
Muscle Toxicities in Childhood Cancer Treatment
Different intramuscular deficiencies have been proposed to mediate the sequelae of post-treatment complications in childhood cancer survivors, including impairment in myofibre progenitor cells (satellite cells), neuromuscular deficiencies and loss of motor units, and mitochondrial dysfunction.22 These various adverse effects are likely caused by specific treatment regimens consisting of one or more components, including multi-agent chemotherapy, radiotherapy, and glucocorticoid treatment.
Chemotherapy
In most childhood cancers, multi-agent chemotherapy constitutes a key component of standard treatment, with numerous agents capable of inducing toxic effects on skeletal muscle. Vincristine is commonly used in the treatment of many malignancies including acute lymphoblastic leukaemia and solid tumours, and is accompanied by severe side effects, including neuropathy and chronic pain.23 The presence of vincristine-induced neuropathy is well-established and observed in both the central nervous system (CNS) and peripheral nervous system (PNS), and is characterised by acute and long-term deficits in both sensory and motor functions lasting several years after cessation of cancer treatment.4,24-34 Vincristine-induced neuropathy occurs as a result of its high binding affinity to β-tubulin, leading to aborted cell division and cell death. The disruption of the β-tubulin assembly and disassembly leads to serious changes in axonal microtubules, causing axonal swelling in myelinated and in unmyelinated fibres, and nerve damage.23 The consequence of these changes has been thoroughly examined through electrophysical examination and studies have reported decreased compound muscle action potential (CMAP),28,34 along with decreased and prolonged latency of motor evoked potentials (MEPs) along the entire motor nervous pathway25 in children receiving treatment. Furthermore, these impairments persist in survivors of childhood cancer, and studies have demonstrated CMAP amplitude less than two standard deviations below normal values27,32 and prolonged latencies of MEP.31 These results strongly indicate that vincristine causes demyelination of motor axons, evidenced by prolonged latencies, and causes damage to motor units leading to denervation of muscle fibres and subsequent muscle atrophy. Collectively, these adverse effects explain in part the acute and prolonged deficits of motor function seen in patients and survivors of childhood cancer,30 whom have received vincristine, even at low doses.4,35
While numerous studies have thoroughly described the neurotoxic impact of vincristine, to our knowledge no studies have evaluated the molecular effects on muscle morphology and intramuscular regulation. Thus, it remains unknown whether vincristine-induced muscle dysfunction is driven exclusively by the impact of impaired neural innervation, or whether vincristine is additionally associated with direct adverse effects on skeletal muscle regulation and metabolism.
Anthracyclines are frequently used in the treatment of childhood cancers and are known to cause cardiovascular complications including cardiomyopathy and endothelial dysfunction.36 While these adverse reactions are considered to be the main drivers of the increased risk of cardiovascular disease in cancer survivors, studies have further outlined a direct anthracycline-related increase in oxidative stress within skeletal muscles contributing to poor physical function, impaired metabolic control, and elevated levels of fatigue.37-38 Muscle biopsies taken before and after a 50-day period of chemotherapy with doxorubicin or melphalan in adults diagnosed with melanoma or sarcoma showed severe reductions in myofibre size, neurogenic alterations, and mitochondria-related damages.39,40
Methotrexate is given intrathecally in childhood cancer cases with CNS involvement, and thus has access to the PNS and CNS. It is generally accepted that methotrexate causes neuropathy, and autopsy studies have reported damage to the myelinated long tracts of the spinal cord, swelling, and loss of axons,41 hypothetically inducing damage and/or loss of motor units, resulting in denervation and subsequently atrophy of muscle fibres.
L-asparaginase is especially used in the treatment of acute lymphoblastic leukaemia as the enzyme reduces the availability of L-asparagine, which is essential for the survival of leukaemic cells. L-asparaginase may inhibit muscle protein synthesis,42 and studies have shown that L-asparaginase treatment is associated with muscle weakness in adolescent and young adult survivors,5 lasting years after cessation of treatment.
Radiotherapy
The risk of adverse events, especially secondary cancer, caused by radiation has hampered the use of radiotherapy in modern paediatric oncology, which applies a risk-adapted approach with the overall intent of minimising or avoiding this modality as much as possible. However, in certain diagnostic groups (e.g. patients with sarcoma) and/or patients who are not responding to chemotherapy,1 radiotherapy remains a treatment option, and is associated with high risk of toxic reaction in the targeted anatomical area. Specifically, radiotherapy may inhibit mitosis of progenitor cells43 and disrupt cell membrane permeability and lipid fluidity, possibly resulting in Na+/K+ pump failure at the neuromuscular junction.44 Furthermore, post-radiation inflammation facilitated by alteration of the growth factor-β family may inhibit muscle growth, and radiation-induced vascular and parenchymal damage may inhibit the supply of nutrients and metabolites.45
Glucocorticoids
Concurrent medication includes glucocorticoids (e.g. prednisolone) as an antiemetic treatment. A supra-physiological concentration of glucocorticoids displays strong immunosuppressive and anti-inflammatory actions, and is essential in the treatment of acute lymphoblastic leukaemia. The use of these agents has traditionally been hampered by considerable metabolic side effects. The adverse reactions to prolonged use of prednisolone in cancer patients is poorly investigated, but it has been demonstrated to cause significant insulin resistance, increased protein breakdown, blunted protein synthesis, and inhibition of insulin signalling in healthy subjects.46
Behavioural factors
Cancer treatment is accompanied by side effects that indirectly affect the skeletal muscle, including: side effects, i.e. nausea (and subsequent malnutrition), pain, reduced pulmonary function, as well as prolonged hospitalisation associated with sedentary behaviour and limited contact with peers. The cumulative effects of the treatment-induced muscle toxicities and physical inactivity augments the level of muscle dysfunction, creating a negative spiral further reducing physical function; in the worst case to the point where patients are no longer functionally independent (Figure 1). Thus, the importance of breaking this vicious cycle is becoming readily apparent.
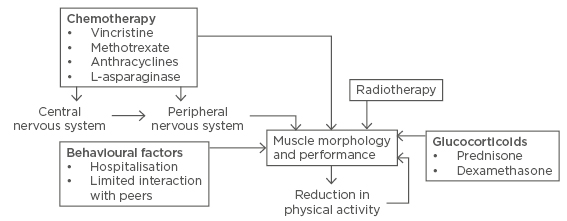
Figure 1: Schematic representation of childhood cancer treatment-related adverse effects on muscle morphology through direct actions or via neurotoxic impairments in the central or peripheral neural system, with a negative impact on muscle morphology and performance, potentially reducing activity level, which in turn reinforces muscular deterioration.
In summary, significant anti-cancer treatment-related muscle toxicities may include myopathies, neuropathy, and intramuscular dysregulation of protein turnover and metabolic properties induced by various therapy components (Table 1). These reactions can lead to decrements in physical function, and subsequent development of metabolic complications which affect cancer survivors long after cessation of treatment; emerging clinical interest is thus directed towards exploring and implementing effective therapeutic countermeasures.
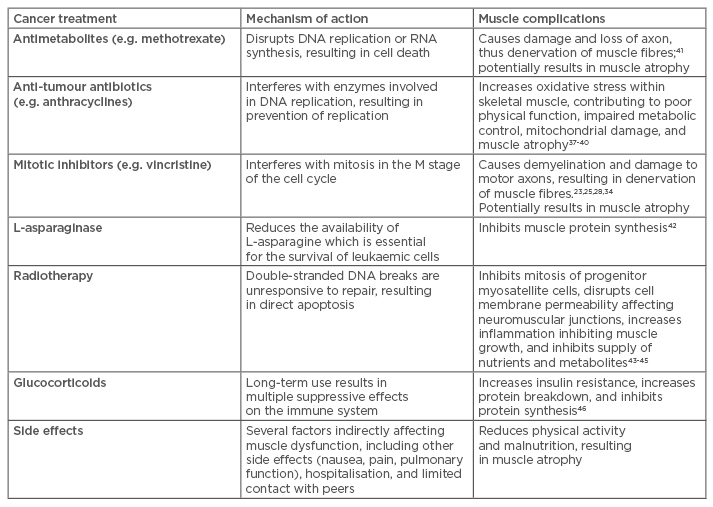
Table 1: Childhood cancer treatments, mechanisms of action, and skeletal muscle complications.
EXERCISE TRAINING IN PAEDIATRIC ONCOLOGY
Exercise training is currently emerging as a promising strategy in the oncology setting, and a growing body of evidence has outlined the beneficial potential of exercise to improve a broad range of physiological endpoints, including muscle-specific outcomes, i.e. muscle strength, lean mass, and mean fibre area. In adults, a plethora of studies have been published showing that cancer patients are capable of performing physical exercise and adapting much to the same extent as non-cancer populations.47,48
While the body of evidence in paediatric oncology is less comprehensive, a number of exercise trials have been performed predominantly in acute lymphoblastic leukaemia patients in the later stages of treatment, demonstrating that exercise is safe and feasible even during intense treatment phases.49 The majority of studies have investigated the effects of exercise interventions on physical function utilising different measures, including timed up and go,50-53 timed up and down stairs,50-54 gait speed test,53,54 and various motor performance batteries.55-57
With emerging safety and feasibility data supporting exercise application, an important next generation of research constitutes mechanistic explorations of exercise-induced improvements with the purpose of developing targeted exercise therapies to reverse treatment-specific muscle toxicities, thus most efficiently ameliorating the level of long-term muscle dysfunction.
Mechanistic Rationale for Structured Exercise Training in Childhood Cancer
Voluntary physical exercise is a pleiotropic strategy inducing homeostatic perturbations in multiple organ systems including skeletal muscle. While the body of mechanistic data is from childhood cancer exercise studies, different plausible candidate mechanisms exist through which exercise may target and counteract treatment specific muscle toxicities.
Resistance exercise to reverse neuromuscular deficits
Treatment-induced neuromuscular deficits are evident through reduced and prolonged latencies of CMAP and MEP observed in childhood cancer patients and survivors.25,27,28,32,34 These alterations may be a result of a demyelination of motor axons, evidenced by the prolonged latencies and/or reduced firing frequencies of motor units and/or damage to motor units. Structured resistance training, as characterised by ‘high load, low volume’ muscle contractions, is known to be highly effective for improving neuromuscular function in different populations.58,59 Resistance training induces neural plasticity and includes alterations of motor unit recruitment, firing frequency, and synchronisation of motor unit activation,58 all of which theoretically would counteract the cancer treatment-induced neuromuscular deficits. Improved firing frequency particularly represents a highly beneficial adaptation to counteract the decline in muscle fibre activation. In addition, increases in firing frequency will improve the ability to produce muscle force at a faster rate, which is of great importance in children suffering from severe neuropathy combined with low thrombocytes and/or porous bones (e.g. some solid tumours), thus lowering the risk of a fall in fragile populations. Furthermore, resistance training can improve markers of metabolic syndrome in healthy children through a combined effect improving insulin sensitivity, blood pressure, lipid profile, and body composition.60
Aerobic exercise to reverse mitochondrial dysfunction
Treatment-induced mitochondrial dysfunction or damage is a result of increased reactive oxygen species (ROS) production, which can lead to muscle atrophy and subsequent weakness.61 Aerobic exercise training, characterised by ‘low load, high volume’ contractions, may counteract this development by stimulating mitochondrial biogenesis and anti-oxidant defences. A key regulator of mitochondrial biogenesis is PGC-1α which is upregulated through prolonged aerobic exercise, increasing the number and size of mitochondria in skeletal muscle62,63 and thus potentially increasing the repair/replacement of damaged mitochondria. Although an increased number of mitochondria in the skeletal muscle would cause the mitochondria to produce more ROS, a parallel regulation of respiratory chain proteins and mitochondrial anti-oxidant enzymes would be an advantage. PGC-1α regulates the expression of anti-oxidant proteins upregulating ROS-removing enzymes, thus improving the anti-oxidant defence.63
In summary, the emergence of exercise-oncology research has led to progressive advances in the application of physical exercise training for patients with cancer, however in the paediatric field a paucity of research initiatives exists. There is a lack of investigations exploring exercise interventions during prolonged hospital stays to break the negative reinforcing spiral of sedentary behaviour and poor physical function, for which there is promising therapeutic potential.
FUTURE DIRECTIONS
Skeletal muscle toxicities may comprise an integrated part of the aetiology related to treatment-induced late effects in childhood cancer survivors. Notably, these impairments may not manifest into clinical symptoms until many years after cessation of treatment, and thus it may be difficult to separate treatment-related from non-treatment-related causes and other derived morbid conditions. To improve the current understanding of the prognostic role of muscle dysfunction in childhood cancer survivors, it is important to investigate the direct molecular impact of common therapeutic modalities in childhood cancer treatment on skeletal muscle biology and regulation. Specific attention should be given to the treatment-induced denervation of muscle fibres and mitochondria dysfunction through muscle biopsy sampling to evaluate fibre type distribution, mean fibre size, satellite cell count, capillary density, and mitochondria content and function.
Furthermore, introducing research initiatives into exercise interventions in paediatric cancer settings is warranted. Specifically, studies investigating the pathophysiological profile of children with cancer undergoing active therapies that may affect exercise adaptations are needed, as well as investigations of the structural and motivational barriers precluding daily activity and exercise for children during prolonged hospitalisation.
In conclusion, a strong clinical rationale is emerging for improving the current understanding of skeletal muscle toxicities in paediatric oncology to advance the evidence base from which therapeutic countermeasures can be developed and implemented in standard childhood cancer care. Maintaining physical capacity through reversal of muscle dysfunction in childhood cancer patients may translate into reduced risk of late-occurring metabolic complications, thus improving the overall quality of childhood cancer survivorship.